Author Response: the Kinesin-5 Tail Domain Directly Modulates the Mechanochemical Cycle of the Motor Domain for Anti-Parallel Microtubule Sliding
openalex(2020)
Abstract
Article Figures and data Abstract Introduction Results Discussion Materials and methods Data availability References Decision letter Author response Article and author information Metrics Abstract Kinesin-5 motors organize mitotic spindles by sliding apart microtubules. They are homotetramers with dimeric motor and tail domains at both ends of a bipolar minifilament. Here, we describe a regulatory mechanism involving direct binding between tail and motor domains and its fundamental role in microtubule sliding. Kinesin-5 tails decrease microtubule-stimulated ATP-hydrolysis by specifically engaging motor domains in the nucleotide-free or ADP states. Cryo-EM reveals that tail binding stabilizes an open motor domain ATP-active site. Full-length motors undergo slow motility and cluster together along microtubules, while tail-deleted motors exhibit rapid motility without clustering. The tail is critical for motors to zipper together two microtubules by generating substantial sliding forces. The tail is essential for mitotic spindle localization, which becomes severely reduced in tail-deleted motors. Our studies suggest a revised microtubule-sliding model, in which kinesin-5 tails stabilize motor domains in the microtubule-bound state by slowing ATP-binding, resulting in high-force production at both homotetramer ends. Introduction Microtubules (MTs) form tracks for the active transport of vesicles and macromolecules inside eukaryotic cells, generate pulling forces during assembly of mitotic spindles, and promote the alignment and segregation of chromosomes (Goshima and Scholey, 2010; Vale, 2003). Fourteen kinesin motor subfamilies utilize MTs as tracks for many diverse functions (Vale, 2003). Among them, kinesin-5 motors represent a unique and highly conserved subfamily that is essential for mitotic spindle assembly during metaphase and spindle elongation during anaphase (Kashina et al., 1996). In contrast to the majority of kinesin classes, kinesin-5 motors adopt a conserved bipolar homotetrameric organization, composed of two dimeric subunits folded in an antiparallel arrangement mediated by the assembly of a 60 nm long central minifilament (Acar et al., 2013; Kashina et al., 1996; Scholey et al., 2014; Singh et al., 2018). Through this conserved bipolar organization, kinesin-5 motors promote MT crosslinking and mediate their sliding apart during mitotic spindle assembly and elongation. This activity can be recapitulated in vitro with purified kinesin-5 motors from a variety of species (Kapitein et al., 2008; Kapitein et al., 2005; van den Wildenberg et al., 2008). Metazoan kinesin-5 motors such as D. melanogaster KLP61F or human Eg5 exhibit slow plus-end directed motility especially during antiparallel MT sliding (Kapitein et al., 2008; Kapitein et al., 2005; Shimamoto et al., 2015; van den Wildenberg et al., 2008). In contrast, yeast kinesin-5 motors, such as Cin8, Kip1 and Cut7 uniquely undergo minus-end directed motility as single motors and reverse direction toward MT plus-ends upon clustering into multi-motor assemblies along single MTs, or during antiparallel MT sliding (Edamatsu, 2014; Fridman et al., 2013; Gerson-Gurwitz et al., 2011; Roostalu et al., 2011; Shapira et al., 2017). The conserved plus-end directed MT sliding activity is essential for mitotic spindle assembly by generating forces exerted on MTs emanating from opposite spindle poles during metaphase and stabilizing the characteristic bipolar spindle organization (Brust-Mascher et al., 2009; Forth and Kapoor, 2017; Subramanian and Kapoor, 2012; Wang et al., 2014). The MT sliding activity is critical for the elongation of mitotic spindles at the midzone region during anaphase (Goshima and Scholey, 2010). Defects in mammalian kinesin-5 motors or their inactivation via inhibitory compounds such as monastrol disrupt the balance of mechanical forces within the spindle and lead to monopolar spindles (Goshima and Scholey, 2010; Goshima et al., 2005). These inhibitory compounds aided in elucidating the fundamental functions of kinesin-5 in mitosis and were suggested to be of therapeutic value in treating rapidly dividing cancer cells (Kwok et al., 2006; Mayer et al., 1999; Owens, 2013). Each kinesin-5 motor consists of a conserved organization including: an N-terminal motor domain, α-helical neck and bipolar assembly regions, and a C-terminal tail domain. The motor domain is connected via a neck-linker to a dimerizing α-helical coiled-coil neck (Turner et al., 2001; Valentine et al., 2006a; Valentine et al., 2006b). The parallel α-helical coiled-coil neck forms part of the 60 nm central antiparallel homotetrameric α-helical minifilament (Acar et al., 2013; Kashina et al., 1996). At the center of this minifilament is a 27 nm antiparallel four α-helical bundle termed the bipolar assembly (BASS) region (Scholey et al., 2014). The bipolar tetrameric organization of the kinesin-5 BASS region orients the two-parallel coiled-coils at the neck and their associated motor domains at an off-set. This results in a 100°-lateral rotation between the two opposite ends that potentially mediates the preference for kinesin-5 to bind and slide two antiparallel MTs (Scholey et al., 2014). A section of unknown structure extends from the C-terminus of the BASS to the tail domain that is located near the motor domains of the antiparallel subunits (Acar et al., 2013). Thus, each end of the kinesin-5 tetramer consists of twin tail and motor domains that emerge from intertwined antiparallel dimeric subunits (Acar et al., 2013; Scholey et al., 2014). The kinesin-5 tail domain contains a conserved BimC box, which is a consensus motif that is phosphorylated by mitotic cyclin-dependent kinases (Blangy et al., 1995; Sharp et al., 1999). Mitotic phosphorylation at the BimC box induces kinesin-5 motors to concentrate along the mitotic midzone where they promote the elongation of the mitotic spindle during late anaphase by sliding apart antiparallel MTs (Sharp et al., 1999), though the role for this phosphorylation in the regulation of kinesin-5 activity remains unknown. Studies of the S. cerevisiae yeast ortholog Cin8 show the tail domain is essential for kinesin-5 function; deletion of the tail leads to a lethal mitotic arrest phenotype in the absence of the analogous motor Kip1 (Hildebrandt et al., 2006). The tail domain of the Xenopus laevis Eg5 has been suggested to form a secondary MT binding site during MT sliding motility (Weinger et al., 2011). However, the exact function of the kinesin-5 tail domain remains unknown. Despite extensive structural, kinetic, and functional analyses of kinesin-5 motors, the origin of the highly conserved MT sliding activity in these motors and its relation the conserved tetrameric organization remains poorly understood. Here, we describe a mechanism for the regulatory motor-to-tail interaction within the homotetrameric kinesin-5 motor and its fundamental role during MT sliding motility. Using biochemical methods, we show that the kinesin-5 tail domain decreases MT-stimulated ATP hydrolysis by binding and stabilizing the motor domain in its ADP or nucleotide-free states. Cryo-EM structures reveal that the KLP61F tail stabilizes the open conformation of the motor by binding its N-terminal subdomain via the α0-helix element located at its tip. We show that human Eg5 motors undergo very slow motility and form clusters along individual MTs, whereas deletion of the tail domain causes Eg5 to undergo rapid motility without clustering along individual MTs. Single-motor fluorescence tracking in MT sliding assays show that Eg5 motors undergo slowed unidirectional motility within active sliding zones, while tail-deleted Eg5 motors undergo rapid motility with frequent switches in direction along the antiparallel MTs within sliding zones. Optical trapping and MT sliding assays reveal that the tail is essential for zippering two MTs into sliding zones through its capacity to generate high pushing forces. In cells, tail-deletion leads to a loss of human Eg5 mitotic spindle localization in mammalian cells while retaining MT binding capability. These studies suggest a revised model for kinesin-5-mediated MT sliding in which the tail domain slows MT-stimulated ATP hydrolysis at each end of the homotetramer and enhance force production essential for MT sliding. Results The kinesin-5 tail domain decreases motor domain MT-stimulated ATP hydrolysis To better understand the role of the tail domain on kinesin-5 function, we first evaluated the effect of the tail domain on MT-stimulated ATP hydrolysis by the kinesin-5 motor domain. To circumvent the added complexity of MT crosslinking and sliding of the full length protein (Figure 1A), we generated constructs consisting of the motor-neck-linker domains (termed ‘motor’; residues 1–356), the isolated tail (termed ‘tail’; residues 913–1056), and a fusion construct in which the motor-neck-linker is linked at its C-terminus to the tail via an 8-residue linker (termed ‘motor-tail fusion’) (Figure 1B–D). We generated and studied both human-Eg5 and Dm-KLP61F constructs in parallel to characterize the conservation of features across these two well-studied orthologs (Table 1; Figure 1B–D; Figure 1—figure supplement 1K–M). For these assays, increasing levels of MTs were added to the motor, to a mixture of motor and tail, or to the motor-tail fusion and resulting MT stimulated ATP hydrolysis rates were measured (Materials and methods; Figure 1B–D). The KLP61F motors robustly hydrolyzed ATP in response to increasing amounts of MTs (kcat7.1 s−1 and Km680 nM; Figure 1B; Table 1). The addition of equimolar KLP61F tail to a mixture of the motor and MTs led to a two-fold decrease in kcat (3.5 s−1 vs 7.1 s−1) but produced little change in Km (756 nM vs 680 nM) suggesting that the tail does not competitively interfere with motor-MT binding but rather modulates MT-stimulated ATP hydrolysis (Figure 1C). The motor-tail fusion exhibited a 2.2-fold decrease in kcat compared to the motor alone (3.4 s−1 vs 7.1 s−1) and a 15-fold decrease in Km compared to that for the motor alone (39 nM vs 680 nM) (Figure 1D). We observed similar behavior for human Eg5 motor and tail constructs. Although, the Eg5 motor displays an eight-fold increase in Km compared to KLP61F motor at 50 mM K-acetate condition (3849 nM vs 680) suggesting the Eg5 motor is slightly sensitive to ionic strength. Therefore, we studied Eg5 constructs at both 20 mM KCl and 50 mM K-acetate ionic strength conditions. At 20 mM KCl condition, the Eg5 tail decreases the MT stimulated Eg5 motor ATP hydrolysis rate by 32% compared to Eg5 motor alone (Kcat7.6 s−1 vs 5.3 s-’;Table 1; Figure 1—figure supplement 1K). The decrease in homogeneous solubility of the Eg5 tail construct compared to the KLP61F tail is likely responsible for this mild decrease in MT-stimulated ATPase. An Eg5 motor-tail fusion construct exhibited a two-fold decrease in MT-stimulated ATPase Kcat (3.47 s−1 vs 7.55 s−1) nearly identical to a parallel construct for Klp61F (Figure 1—figure supplement 1L). The tail-induced decrease in MT-stimulated ATPase activity, however, was somewhat reduced at a 50 mM K-acetate condition (5.71 s−1 vs 6.81 s−1; Table 1; Figure 1—figure supplement 1M), suggesting that in this condition the tail-to-motor regulation is weakened (see next section). Our studies reveal that the kinesin-5 tail domain decreases the MT-stimulated ATP hydrolysis rate of the motor domain in either an isolated or a fused configuration and that this feature is conserved across human Eg5 and Dm-KLP61F. Figure 1 with 1 supplement see all Download asset Open asset The kinesin-5 tail domain inhibits the motor domain MT-stimulated ATPase activity through stabilization of the MT bound nucleotide-free state. (A) Top, domain organization for the kinesin-5 motors, Dm KLP61F and Hs FL-Eg5 consisting of conserved N-terminal motor domain, central BASS domain and C-terminal tail domain. Bottom, homotetrameric organization of kinesin-5. (B) Steady-state MT-stimulated ATP hydrolysis for KLP61F motor. (N = 2) (C) Steady state MT stimulated ATP hydrolysis for equimolar KLP61F motor + tail constructs. (N = 2) (D) Steady state MT stimulated ATP hydrolysis for KLP61F motor-tail fusion. (N = 2) (E) Affinity co-purification of the KLP61F motor using the KLP61F tail-StrepII trapping one of three nucleotide states conditions. Top panel, SDS-PAGE for each condition: 5 μmol motor + tail mixture is loaded onto Streptactin XT resin (Load), flow-through fraction (FL) and biotin elution fraction (elute) are shown. Left lanes, incubation of motor + tail with non-hydrolysable ATP-analog, 2 mM AMPPNP. Center lanes, incubation of motor + tail with 2 mM ADP. Right lanes, incubation of motor + tail in the presence of 2 U Apyrase resulting in a nucleotide free state. Bottom panel, quantitative densitometry reveals the molar ratios of motor to tail in the elution fractions at 25 mM KCl (yellow column) and 75 mM KCl (blue columns) buffer conditions (N = 3, n = 6 for each column). (F) MT co-sedimentation assays of the KLP61F motor (motor) and tail domain (tail) with MTs (MT) in the presence of non-hydrolysable analog, 2 mM AMPPNP at 25 mM KCl. Co-sedimentation was carried out with MTs for 0.01, 0.025, 0.05, 0.1,0.25 μmol motor and tail mixture at 25 mM KCl conditions, and in the absence of MTs (-MT) control. Additional data are shown in Figure 1—figure supplement 1B. (G) MT co-sedimentation assays of the KLP61F motor (motor) and tail domain (tail) with MTs (MT) in the presence of 2 mM ADP at 25 mM KCl. Co-sedimentation was carried out with MTs for 0.01, 0.025, 0.05, 0.1,0.25 μmol motor and tail mixture at 25 mM KCl condition and in the absence of MTs (-MT) control. Additional data are shown in Figure 1—figure supplement 1D. (H) MT co-sedimentation assays of the KLP61F motor (motor) and tail domain (tail) with MTs (MT) in the nucleotide-free state, achieved by adding 10 U of Apyrase. Co-sedimentation was carried out with MTs for 0.01, 0.025, 0.05, 0.1,0.25 μmol motor and tail mixture at 25 mM KCl conditions and in the absence of MTs (-MT) control. Detailed and additional data are shown in Figure 1—figure supplement 1E. (I) Molar ratios of motor to tubulin monomer (blue) and tail to tubulin dimer (green) measured using quantitative densitometry (Materials and methods). The motor-to-tubulin ratios observed at 75 mM KCl are roughly 0.5 and the amount of tail bound to the motor increases in the ADP and Nucleotide-free states increases in the 25 mM KCl compared to the 75 mM KCl conditions. The amount of tail bound to motor remain low in the AMPPNP and ADP.AlF4 states compared to the ADP and nucleotide-free states. (J) Summary of data presented resulting in a model for motor (green) and tail (blue) domain affinities and MT (blue and pink) binding capacities in four nucleotide states during the ATP hydrolysis cycle. Table 1 Steady kinetic parameters for MT-activated ATP hydrolysis. ConstructSourceIonic Strengthkcat (sec−1)K0.5,MT (nM)MotorDm KLP61F50 mM K Acetate7.1 ± 0.1680 ± 48Motor + TailDm KLP61F50 mM K Acetate3.5 ± 0.5757 ± 327Motor-Tail fusionDm KLP61F50 mM K Acetate3.3 ± 0.139 ± 11MotorHs Eg520 mM KCl7.3 ± 0.2334 ± 14Motor-Tail fusionHs Eg520 mM KCl3.4 ± 0.2158 ± 41Motor + TailHs Eg520 mM KCl5.4 ± 0.3209 ± 56MotorHs Eg550 mM K Acetate6.71 ± 0.73849 ± 800Motor-Tail fusionHs Eg550 mM K Acetate5.87 ± 0.23391 ± 59 The tail domain binds the motor domain in the ADP or nucleotide-free states To understand the biochemical basis for the kinesin-5 tail-mediated regulation of motor domain MT-stimulated ATP hydrolysis, we studied the binding of kinesin-5 motor domain with and without the MT lattice. First, we measured the capacity of the motor domain to co-purify and co-elute with a C-terminally strep-II tagged KLP61F tail domain construct onto streptactin XT resin (See Materials and methods; Figure 1E; Figure 1—figure supplement 1A). We compared the binding activities at two ionic strength conditions (25 and 75 mM KCl) for which motility assays indicated distinct modes of tail-dependent motility regulation (see below; Figures 3–4). We also tested how the binding is influenced by distinct nucleotides that trap the motor domain in different steps of the ATP hydrolysis cycle (Figure 1E). At 25 mM KCl, the motor co-eluted with the tail in the 2 mM ADP and in the nucleotide free state (in presence of Apyrase enzyme) but very poorly co-eluted with the tail in the presence of 2 mM AMPPNP (Figure 1E). Furthermore, the proportion of Klp61F motor co-eluting with the tail decreased dramatically at 75 mM KCl conditions (Figure 1E). Higher concentration of motor and tail did not change the molar ratio of the eluting proteins (Figure 1—figure supplement 1A). Next, we reconstituted binding of the Klp61F and Eg5 motor and tail constructs onto Paclitaxel-stabilized MTs in different nucleotide state conditions and analyzed the binding using MT co-sedimentation assays (Figure 1F–I; Figure 1—figure supplement 1B–H). We again used conditions that mimic each step of the kinesin ATP hydrolysis cycle (Figure 1F–I; Figure 1—figure supplement 1B–H). We also compared the binding activities at two ionic strength conditions (25 and 75 mM KCl) as described above. The KLP61F tail and motor individually bound MTs in the presence of 2 mM ADP, as has been shown previously (Figure 1—figure supplement 1G–H; Weinger et al., 2011). The molar ratio of the bound tail and motor to MTs (polymerized tubulin) are roughly ~0.5 and~1 per αβ-tubulin at 25 mM KCl and decreased at 75 mM KCl (Figure 1—figure supplement 1F,H). MT co-sedimentation of motor and tail together in the presence of 2 mM AMPPNP or ADP.AlF4 revealed that the tail is displaced from MTs, while the motor bound with high affinity to saturation (Figure 1F; Figure 1—figure supplement 1B–C). Even at the lowest molar ratio of motor to MTs, where there is an abundance of unoccupied MT lattice sites, the tail does not co-sediment with MTs and does not compete with the motor for MT binding sites. In the ADP and nucleotide free states, the KLP61F motor domain recruits the tail domain more effectively to the MT lattice despite the different motor affinities for MTs. In the presence of 2 mM ADP, the motor binds MTs with low affinity in a concentration-dependent manner, and its binding molar ratio is higher at 25 mM KCl compared to 75 mM KCl (Figure 1G; Figure 1—figure supplement 1D). At 25 mM KCl, a higher amount of motor binds MTs, resulting in a higher amounts of tail being recruited into the pellet, approaching a molar ratio of ~1 motor per αβ-tubulin MT lattice site (Figure 1G; Figure 1—figure supplement 1D). At 75 mM KCl, both motor and tail amounts bound to MTs decreased and their molar ratio decreased (Figure 1I). MT co-sedimentation in the presence of apyrase (1 U/ml) to promote thenucleotide-free state of the motor revealed a high affinity of the motor for MTs at both 25 and 75 mM KCl (Figure 1H). Under the nucleotide-free condition, higher levels of tail are recruited in a manner that correlates with the amount of motor bound to MT in the pellet fraction (Figure 1I). Quantitative densitometry analyses indicates the molar ratios of tail recruited to the MT-bound fraction are highest in the ADP and nucleotide-free state in contrast to the AMPPNP and ADP.AlF4 states (Figure 1I). We studied analogous human Eg5 motor and tail constructs in the AMPPNP, ADP, and nucleotide free states which revealed essentially similar patterns of behavior to those described above for KLP61F (Figure 1—figure supplement 1I–J). The human Eg5 motor recruits the Eg5 tail to the MT-bound fraction in the presence of 2 mM ADP. Despite the high affinity of the Eg5 motor for MTs in the presence of 2 mM AMPPNP, the tail remains unbound and in the soluble fraction (Figure 1—figure supplement 1H–J). This suggest that the Eg5 tail binds to Eg5 motor in the presence of 2 mM ADP, but its binding to the Eg5 motor in presence of 2 mM AMPPNP is similar but weaker than the KLP61F tail (Figure 1—figure supplement 1I–J). Together, our studies revealed that the ATP and ADP.Pi states of the kinesin-5 motor domains exhibit a high binding affinity for MTs, but a low affinity for the tail domain, while also displacing the isolated tail from MTs. In contrast, in the ADP and nucleotide-free states the kinesin-5 motor exhibits increased affinity for the tail, leading the tail to be recruited to the MT bound fraction despite the difference in the motor-MT affinity in these two states (Figure 1J). Cryo-EM structure reveals the tail domain stabilizes an open active site conformation of the motor domain We used cryo-electron microscopy (cryo-EM) to investigate the kinesin-5 tail-motor interface and its role in motor mediated ATP hydrolysis. We collected cryo-EM images of KLP61F motor decorated onto MTs in the AMPPNP state, and the KLP61F motor and tail decorated onto MTs in the nucleotide free state. Class averages for the MT-decorated segments in the two states clearly revealed repeating motordensities decorating the MT lattice sites (Figure 2E). New and previously described image analysis strategies (see Materials and methods) were used to calculate and refine structures of MTs decorated with the KLP61F motor in the AMPPNP state and the KLP61F motor and tail complex in the nucleotide-free state to ~4.4 Å. We adopted a new strategy to apply local classification to the motor and tail densities in the nucleotide free state, which involved classifying small patch of the MT lattice (Materials and methods). Only 70% of the data were utilized for this refinement step (Materials and methods). However, this new strategy generally improved the resolution of the motor domain density but did not enhance the occupancy or resolution for the tail (Figure 2A–F; Figure 2—figure supplement 1A–C; Table 2; see Materials and methods). Additional density, which we attribute to the tail domain, is observed on top of the motor domain in the nucleotide-free motor and tail-decorated MTs. Despite the size of the tail domain (80–100 residues), this density is small and accounts for only about one third of this mass (Figure 2—figure supplement 1B–C,H). This suggests that two thirds of the tail is either unstructured, or forms a flexibly attached and separate domain; a major part of the tail region is rendered invisible after averaging. Furthermore, the density attributed to the tail region cannot be interpreted in terms of secondary structure as there is a substantial drop in the resolution to ~8 Å at the motor-tail interface (Figure 2—figure supplement 1B–C; Video 1). In keeping with the biochemical data, we do not observe any ordered additional density on the MT lattice, consistent with the idea that the tail does not bind the MT lattice in the presence of the motor domain. Overall, the data strongly suggest that the tail interacts loosely with the underlying motor domain, binding directly to its α0 helix hairpin element, located on its MT minus end facing side (Figure 2D–F). Comparison of the KLP61F motor AMPPNP structure with the motor-tail nucleotide-free structure revealed conformational changes within the motor domain leading a major rotation in the α0 helix hairpin (Figure 2D–F; Video 1), and suggests how elements of the nucleotide-free state form an effective binding site for the tail (Figure 2C). One end of the α0 helix is connected to the phosphate binding loop (p-loop) (Figure 2G) and allows possible feedback between the nucleotide pocket and the tail binding site. Figure 2 with 1 supplement see all Download asset Open asset Cryo-EM reveals the kinesin-5 tail engages the motor domain in the nucleotide-free state and stabilizes an open ATP active site. (A-C) (A) Side view of 4.0 Å cryo-EM structure of Klp61F motor domain decorated MT unit in the AMPPNP state. A single kinesin motor-bound αβ-tubulin unit is shown. The segmented motor domain (yellow) and α-tubulin (cyan) and β-tubulin (green) densities are sown. (B) De novo built KLP61F motor domain model in the AMPPNP state (red) displayed within the motor domain density. The αβ-tubulin dimer model fitted into the αβ-tubulin density (green and cyan). (C) Top end view of the kinesin-5 motor density map with the MT density computationally removed. (D-F) (D) Side view of 4.0 Å cryo-EM structure of Klp61F motor and tail domains decorated onto MTs in the nucleotide-free state obtained using well-established and refined with new strategies (Figure 2—figure supplement 1A–C; see Materials and methods). The kinesin-motor + tail bound to a single αβ-tubulin unit is shown. Segmentation of the motor domain (yellow), tail domain is shown (blue), α-tubulin (cyan) and β-tubulin (green). (E) De novo built Klp61F motor domain model in the nucleotide-free state (orange) displayed within the motor domain density and the tail density (dark blue). The αβ-tubulin dimer fitted into the αβ-tubulin density (green and cyan). (F) Top-end view of the docked kinesin-5 motor and tail density with the MT density computationally removed. (G) Conformational transition of Klp61F motor domain from nucleotide-free (light gray) to AMPPNP (dark gray). The elements that undergo the most change in colors: α6 (blue), α2 (yellow), p-loop (green) and α0 helix (red). (I-J) Two views of the Klp61F AMPPNP and nucleotide-free motor domain models describing the movements of motor with N-terminal subdomain (deep blue, nucleotide-free (nuc.-free); light blue, AMPPNP) and Upper subdomain (deep pink, nucleotide-free; light pink, AMPPNP) around the α4-helix, L11 switch II (swII) MT bound subdomain (deep red, nucleotide-free; light red, AMPPNP). The switch I (swI) changes conformation in response to ATP binding. The N-terminal subdomain rotation leads to a 20° rotation of the α0-helix closer to the MT surface in the AMPPNP state. The tail binds the α0-helix in the nucleotide-free state. H) Side-view of the Klp61F motor domain maps in AMPPNP (red) compared to the nucleotide-free state model (orange) docked into the nucleotide free-state motor density with the tail density shown in blue. Table 2 Cryo-EM KLp61F motor and tail MT structures: collection and reconstruction. Dm Klp61F motor-AMPPNP (15 protofilaments)Dm Klp61F- motor AMPPNP (14 protofilaments)Dm KLP61F motor-tail- nucleotide free (15 protofilaments)Dm Klp61F5 motor-tail-nucleotide free (14-protofilaments)Data collectionMicroscopeTitan Krios (FEI)Titan Krios (FEI)Titan Krios (FEI)Titan Krios (FEI)Voltage (kV)300300300300Ls22,500X22,500X22,500X22,500XCumulative exposure dose (e- Å−2)38384040Exposure rate (e-/pixel/sec)7.97.98.38.3DetectorK2 SummitK2 SummitK2 SummitK2 SummitPixel size (Å)*1.311.311.311.31Defocus range (µm)0.3–3.780.7–3.780.19–5.120.19–5.12Average defocus (m)1.751.751.861.86Micrographs Used12601260955955Total extracted helical segment (no.)73,45173,45144,08144,081Refined helical segment (no.)21,00439,001949027,433ReconstructionFinal helical segments (no.)21,00439,220949014,570Symmetry imposedHPHPHPHPResolution (global) FSC 0.1434.24.44.24.3 Video 1 Download asset This video cannot be played in place because your browser does support HTML5 video. You may still download the video for offline viewing. Download as MPEG-4 Download as WebM Download as Ogg Structural transition of the kinesin-5 motor domain from AMPPNP to nucleotide state and its effect on binding of the tail domain. View of the kinesin-5 motor domain map with AMPPNP showing the motor domain model, transition to the motor nucleotide state map showing the site of binding for the tail domain density, and model for the motor. Views of the two states using three motor subdomains and their conformational changes in the N-terminal subdomain (blue) and its effect on the ATP binding site and the rotation around the Upper subdomain (pink) and the MT bound subdomain (red). Model building for both the kinesin-5 AMPPNP and nucleotide-free motor domain structures revealed conformational changes in α0-helix hairpin that may regulate the affinity of the tail within the motor domain (Table 3; Figure 2C,F; Video 1). Compared to kinesin-1, the kinesin-5 motor structure revealed the conserved kinesin fold with two longer and reorganized class-specific L6 and L8 loops which form part of the motor MT-binding interface (Figure 2—figure supplement 1E–F-G), similar to the map of the recently-determined S. pombe and U. maydis kinesin-5 motor domain structures (von Loeffelholz and Moores, 2019; von Loeffelholz et al., 2019). Structurally, the kinesin-5 motor domain can be divided to three subdomains, comparable to those seen in kinesin-1: the N-terminal subdomain, the upper subdomain, and the MT-binding subdomain (Figure 2FH-I; Cao et al., 2014; Shang et al., 2014). The MT-bound subdomain consists of the α4-helix L11 and L12, as described for kinesin-1 (Figure 2I–J; Figure 2—figure supplement 1E–F-G; Video 1). As in kinesin-1, the N-terminal subdomain rotates around the MT-bound subdomain in the kinesin-5 nucleotide-free state, leading to a reorganization of the MT minus-end facing end of the motor (Figure 2H–I; Figure 2—figure supplement 1F–G). In the nucleotide-free state, the N-terminal subdomain (blue) rotates upward with respect to the upper subdomain (pink) and the MT bound α4 helix (switch-II), and L11 (red) to open the switch I loop in the active site (Figure 2H–J). Compared to the AMPPNP state, the N-terminal subdomain in the nucleotide-free state rotates upward by 20° causing the α0-helix hairpin, which lies at its extreme tip, to move upward by 10 Å from the MT surface (Figure 2H–J; Video 1). This N-terminal subdomain rotation repositions the switch I, switch II, and P-loops, resulting in an open active site (Figure 2H–J), whereas in the AMPPNP state, the α0-helix is
MoreTranslated text
Key words
Microtubules,Catalytic Nanomotors
AI Read Science
Must-Reading Tree
Example
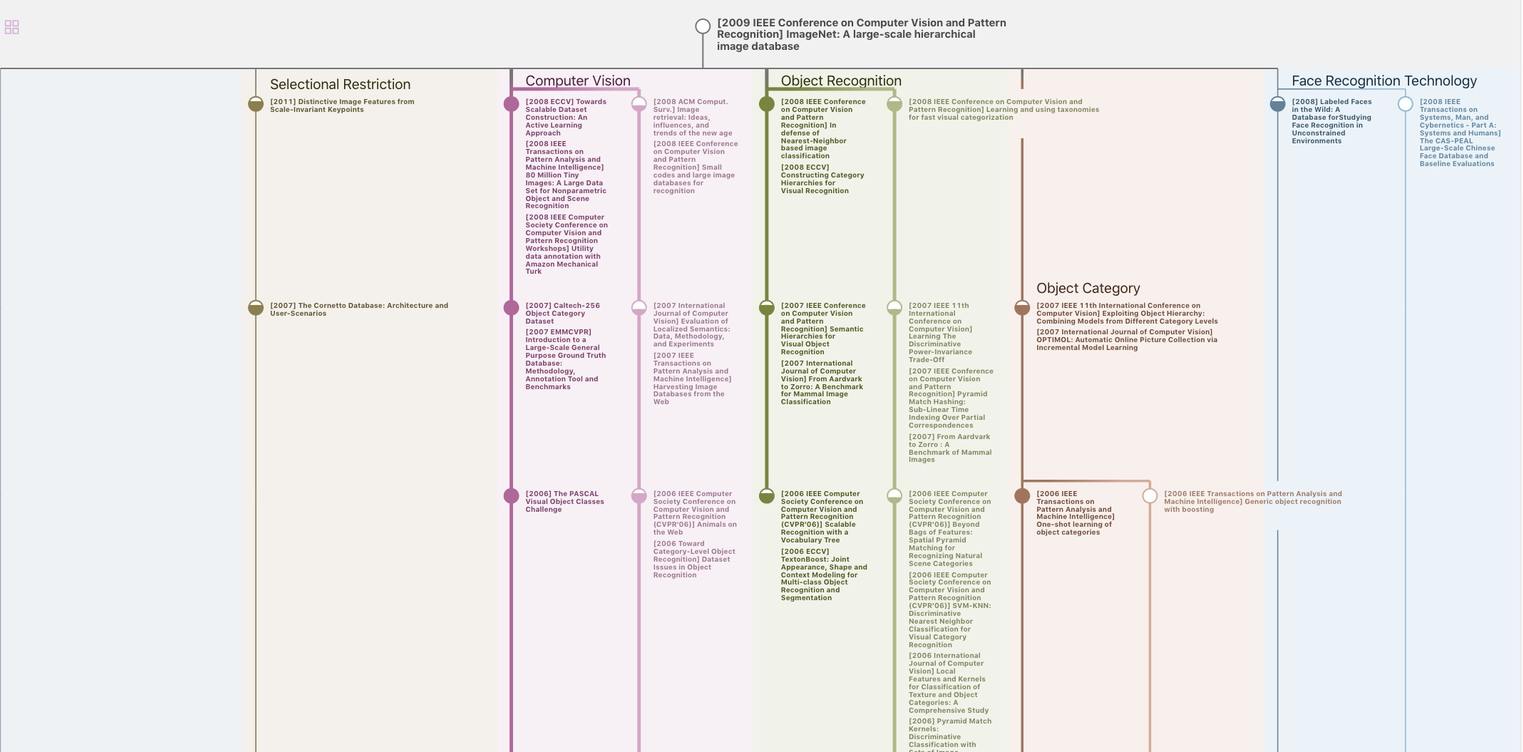
Generate MRT to find the research sequence of this paper
Chat Paper
Summary is being generated by the instructions you defined