Mip6 binds directly to the Mex67 UBA domain to maintain low levels of Msn2/4 stress‐dependent mRNAs
EMBO Reports(2019)
摘要
Article3 November 2019free access Transparent process Mip6 binds directly to the Mex67 UBA domain to maintain low levels of Msn2/4 stress-dependent mRNAs Manuel Martín-Expósito orcid.org/0000-0002-0852-009X Gene Expression and RNA Metabolism Laboratory, Instituto de Biomedicina de Valencia (CSIC), Valencia, Spain Gene Expression and RNA Metabolism Laboratory, Centro de Investigación Príncipe Felipe (CIPF), Valencia, Spain Search for more papers by this author Maria-Eugenia Gas Gene Expression and RNA Metabolism Laboratory, Centro de Investigación Príncipe Felipe (CIPF), Valencia, Spain Search for more papers by this author Nada Mohamad Signal Transduction Laboratory, Instituto de Biomedicina de Valencia (CSIC), Valencia, Spain Search for more papers by this author Carme Nuño-Cabanes Gene Expression and RNA Metabolism Laboratory, Instituto de Biomedicina de Valencia (CSIC), Valencia, Spain Gene Expression and RNA Metabolism Laboratory, Centro de Investigación Príncipe Felipe (CIPF), Valencia, Spain Search for more papers by this author Ana Tejada-Colón Gene Expression and RNA Metabolism Laboratory, Instituto de Biomedicina de Valencia (CSIC), Valencia, Spain Search for more papers by this author Pau Pascual-García Gene Expression and RNA Metabolism Laboratory, Centro de Investigación Príncipe Felipe (CIPF), Valencia, Spain Search for more papers by this author Lorena de la Fuente Genomics of Gene Expression Laboratory, Centro de Investigación Príncipe Felipe (CIPF), Valencia, Spain Search for more papers by this author Belén Chaves-Arquero orcid.org/0000-0002-2761-2336 Department of Biological Physical Chemistry, Institute of Physical-Chemistry “Rocasolano” (CSIC), Madrid, Spain Search for more papers by this author Jonathan Merran Department of Molecular Biology and Genetics, Johns Hopkins University School of Medicine, Baltimore, MD, USA Search for more papers by this author Jeffry Corden Department of Molecular Biology and Genetics, Johns Hopkins University School of Medicine, Baltimore, MD, USA Search for more papers by this author Ana Conesa Genetics Institute, University of Florida, Gainesville, FL, USA Microbiology and Cell Science Department, Institute for Food and Agricultural Research, University of Florida, Gainesville, FL, USA Search for more papers by this author José Manuel Pérez-Cañadillas Department of Biological Physical Chemistry, Institute of Physical-Chemistry “Rocasolano” (CSIC), Madrid, Spain Search for more papers by this author Jerónimo Bravo Signal Transduction Laboratory, Instituto de Biomedicina de Valencia (CSIC), Valencia, Spain Search for more papers by this author Susana Rodríguez-Navarro Corresponding Author [email protected] orcid.org/0000-0001-7472-3111 Gene Expression and RNA Metabolism Laboratory, Instituto de Biomedicina de Valencia (CSIC), Valencia, Spain Gene Expression and RNA Metabolism Laboratory, Centro de Investigación Príncipe Felipe (CIPF), Valencia, Spain Search for more papers by this author Manuel Martín-Expósito orcid.org/0000-0002-0852-009X Gene Expression and RNA Metabolism Laboratory, Instituto de Biomedicina de Valencia (CSIC), Valencia, Spain Gene Expression and RNA Metabolism Laboratory, Centro de Investigación Príncipe Felipe (CIPF), Valencia, Spain Search for more papers by this author Maria-Eugenia Gas Gene Expression and RNA Metabolism Laboratory, Centro de Investigación Príncipe Felipe (CIPF), Valencia, Spain Search for more papers by this author Nada Mohamad Signal Transduction Laboratory, Instituto de Biomedicina de Valencia (CSIC), Valencia, Spain Search for more papers by this author Carme Nuño-Cabanes Gene Expression and RNA Metabolism Laboratory, Instituto de Biomedicina de Valencia (CSIC), Valencia, Spain Gene Expression and RNA Metabolism Laboratory, Centro de Investigación Príncipe Felipe (CIPF), Valencia, Spain Search for more papers by this author Ana Tejada-Colón Gene Expression and RNA Metabolism Laboratory, Instituto de Biomedicina de Valencia (CSIC), Valencia, Spain Search for more papers by this author Pau Pascual-García Gene Expression and RNA Metabolism Laboratory, Centro de Investigación Príncipe Felipe (CIPF), Valencia, Spain Search for more papers by this author Lorena de la Fuente Genomics of Gene Expression Laboratory, Centro de Investigación Príncipe Felipe (CIPF), Valencia, Spain Search for more papers by this author Belén Chaves-Arquero orcid.org/0000-0002-2761-2336 Department of Biological Physical Chemistry, Institute of Physical-Chemistry “Rocasolano” (CSIC), Madrid, Spain Search for more papers by this author Jonathan Merran Department of Molecular Biology and Genetics, Johns Hopkins University School of Medicine, Baltimore, MD, USA Search for more papers by this author Jeffry Corden Department of Molecular Biology and Genetics, Johns Hopkins University School of Medicine, Baltimore, MD, USA Search for more papers by this author Ana Conesa Genetics Institute, University of Florida, Gainesville, FL, USA Microbiology and Cell Science Department, Institute for Food and Agricultural Research, University of Florida, Gainesville, FL, USA Search for more papers by this author José Manuel Pérez-Cañadillas Department of Biological Physical Chemistry, Institute of Physical-Chemistry “Rocasolano” (CSIC), Madrid, Spain Search for more papers by this author Jerónimo Bravo Signal Transduction Laboratory, Instituto de Biomedicina de Valencia (CSIC), Valencia, Spain Search for more papers by this author Susana Rodríguez-Navarro Corresponding Author [email protected] orcid.org/0000-0001-7472-3111 Gene Expression and RNA Metabolism Laboratory, Instituto de Biomedicina de Valencia (CSIC), Valencia, Spain Gene Expression and RNA Metabolism Laboratory, Centro de Investigación Príncipe Felipe (CIPF), Valencia, Spain Search for more papers by this author Author Information Manuel Martín-Expósito1,2,‡, Maria-Eugenia Gas2,‡, Nada Mohamad3,‡, Carme Nuño-Cabanes1,2, Ana Tejada-Colón1, Pau Pascual-García2,†, Lorena Fuente4, Belén Chaves-Arquero5, Jonathan Merran6, Jeffry Corden6, Ana Conesa7,8, José Manuel Pérez-Cañadillas5, Jerónimo Bravo3 and Susana Rodríguez-Navarro *,1,2 1Gene Expression and RNA Metabolism Laboratory, Instituto de Biomedicina de Valencia (CSIC), Valencia, Spain 2Gene Expression and RNA Metabolism Laboratory, Centro de Investigación Príncipe Felipe (CIPF), Valencia, Spain 3Signal Transduction Laboratory, Instituto de Biomedicina de Valencia (CSIC), Valencia, Spain 4Genomics of Gene Expression Laboratory, Centro de Investigación Príncipe Felipe (CIPF), Valencia, Spain 5Department of Biological Physical Chemistry, Institute of Physical-Chemistry “Rocasolano” (CSIC), Madrid, Spain 6Department of Molecular Biology and Genetics, Johns Hopkins University School of Medicine, Baltimore, MD, USA 7Genetics Institute, University of Florida, Gainesville, FL, USA 8Microbiology and Cell Science Department, Institute for Food and Agricultural Research, University of Florida, Gainesville, FL, USA †Present address: Department of Cell and Developmental Biology, Epigenetics Institute, Perelman School of Medicine, University of Pennsylvania, Philadelphia, PA, USA ‡These authors contributed equally to this work *Corresponding author. Tel: +34 963391757; E-mail: [email protected] EMBO Rep (2019)20:e47964https://doi.org/10.15252/embr.201947964 PDFDownload PDF of article text and main figures. Peer ReviewDownload a summary of the editorial decision process including editorial decision letters, reviewer comments and author responses to feedback. ToolsAdd to favoritesDownload CitationsTrack CitationsPermissions ShareFacebookTwitterLinked InMendeleyWechatReddit Figures & Info Abstract RNA-binding proteins (RBPs) participate in all steps of gene expression, underscoring their potential as regulators of RNA homeostasis. We structurally and functionally characterize Mip6, a four-RNA recognition motif (RRM)-containing RBP, as a functional and physical interactor of the export factor Mex67. Mip6-RRM4 directly interacts with the ubiquitin-associated (UBA) domain of Mex67 through a loop containing tryptophan 442. Mip6 shuttles between the nucleus and the cytoplasm in a Mex67-dependent manner and concentrates in cytoplasmic foci under stress. Photoactivatable ribonucleoside-enhanced crosslinking and immunoprecipitation experiments show preferential binding of Mip6 to mRNAs regulated by the stress-response Msn2/4 transcription factors. Consistent with this binding, MIP6 deletion affects their export and expression levels. Additionally, Mip6 interacts physically and/or functionally with proteins with a role in mRNA metabolism and transcription such as Rrp6, Xrn1, Sgf73, and Rpb1. These results reveal a novel role for Mip6 in the homeostasis of Msn2/4-dependent transcripts through its direct interaction with the Mex67 UBA domain. Synopsis The RNA binding protein Mip6 interacts with the ubiquitin-associated domain of Mex67 and attenuates the nuclear export of mRNAs regulated by the stress response Msn2/4 transcription factors. The key elements involved in Mip6/Mex67 interaction are a loop in Mip6 RRM4 containing tryptophan 442, and helix H2 of the Mex67 UBA domain. Mip6 binds to Msn2/4-regulated transcripts attenuating their expression and export under non-stress. Mip6 shuttles between the nucleus and the cytoplasm in a Mex67-dependent manner and interacts with Rpb1, Sgf73, Rrp6 and Xrn1 along the gene expression pathway. Introduction mRNA export from the nucleus is a crucial step in gene expression in order to ensure efficient protein production. In eukaryotic cells, mRNA export is connected to upstream and downstream events that modulate mRNA fate through the action of a repertoire of factors and multiprotein complexes 1-4. Among these factors, the yeast export receptor heterodimer Mex67-Mtr2 (TAP-p15 in humans), which is loaded onto transcripts early during transcription 5, 6, is crucial for mRNA export, conferring competency on messenger ribonucleoparticles (mRNPs) for docking at the nuclear pore complex (NPC). In addition to Mex67-Mtr2, mRNPs incorporate adaptor proteins that modulate mRNA metabolism and that can act positively or negatively in mRNA export 7-9. The best known of these proteins are the RNA-binding proteins Nab2, Hrb1, Gbp2, and Npl3 that interact with Mex67-Mtr2 10, . Furthermore, some RNA-binding proteins that are also known as “guard proteins” can bind to pre-mRNA, acting as a tag of the unprocessed state 13 and preventing undesirable Mex67 contacts, thereby functioning as a mechanism for blocking uncontrolled RNA export 10, 14, 15. This quality control mechanism might also be used to regulate export routes under specific circumstances such as stress during cellular growth. Cells have evolved a sophisticated stress response that includes a specific gene expression program 16-19. Under stress, splicing of most housekeeping genes is disrupted and their export to the cytoplasm is blocked 16, 18. Simultaneously, the export and translation of stress-responsive genes is enhanced, and their increased expression becomes essential for cell survival 20, 21. Recent work has investigated the role of “guard proteins” in controlling Mex67 binding to housekeeping and stress-responsive transcripts upon stress 15. These data showed that Mex67 is directly recruited to stress-responsive transcripts through interaction with the transcription factor Hsf1 at early steps during transcription. This recruitment is independent of adaptor proteins and facilitates the export of these transcripts, bypassing the quality control mechanisms. However, such independence from adaptor (or guard) proteins that is observed for Hsf1 targets might not be universal for all types of stress-responsive mRNAs and furthermore cannot be assumed for the variety of stresses in which Hsf1 is not directly involved. An example of the latter case is Msn2/4 transcription factor-dependent transcription 15 that is involved in the environmental stress response (ESR) that senses different kinds of stresses including heat shock, glucose starvation, and oxidative and osmotic stress 22-25. Binding of Msn2/4 to specific DNA sequences leads to a transcriptional change that mediates cell survival 26, 27. Whether Msn2/4 transcripts are independent of adaptor proteins or whether they require specific factors for their metabolism is currently unknown. Mip6 (Mex67 Interacting Protein 6) was discovered in 1997 during a yeast two-hybrid (Y2H) screen using Mex67 as bait 28. This putative RNA-binding protein bears four RRMs and was suggested by Hurt and colleagues to interact with the C-terminal domain of Mex67. However, it is only recently that further functional characterization of Mip6 was performed, when the role of this factor in different fields started to attract attention. In a recent screen in yeast for dosage-sensitive proteins that have a propensity for liquid–liquid phase separation (LLPS), it was found that, at least for Mip6, liquid–liquid demixing is associated with toxicity when the protein is highly overexpressed 29. Under these conditions, Mip6 changes its cellular localization and accumulates into liquid-like cytoplasmic foci. Apart from increasing interest in understanding the physiological consequences of liquid–liquid demixing in the cell, this study did not address the functional role of Mip6 in yeast. A more recent study proposed a specific role for Mip6 during sporulation 30. Mip6, together with its paralogue Pes4, was suggested to act as regulators of late translation, protection, and mRNA localization within the NDT80 regulon that are translated at different times during sporulation. In this work, we showed that Mip6 interacts functionally and/or physically with factors involved in several steps of mRNA metabolism such as Mex67, Rrp6, Xrn1, Sgf73, and Rpb1 and we further characterized its interaction with the general exporter Mex67 both at functional and at structural levels. The functional and physical interaction between Mip6 and these factors has been characterized by using a multidisciplinary approach, combining biophysical, biochemical, molecular, and microscopical techniques. The data show that Mip6 and Mex67 interact directly and that this interaction is important for Mip6 in vivo function, which is related to the Msn2/4 regulatory network attenuating the expression of their target genes under non-stress. These data indicate that Mip6 is a new regulator of stress responses. Results Mip6 interacts with key components of the mRNA export machinery In searching for novel interacting partners of Sus1, we purified Sus1 in the absence of Ubp8, when it is preferentially associated only with the deubiquitination module (DUB) and with TREX2 31. In addition to expected factors, we found that the putative RNA-binding protein Mip6 co-purified with Sus1-TAP (Fig 1A). Mip6 was first reported as the Mex67 Interacting Protein 6 (MIP6) in a two-hybrid screen using Mex67 as a bait 28. We further confirmed that Mip6 co-purified with Mex67 from yeast cells (Fig 1B) and that this interaction is not affected by RNase A treatment (Fig 1C), indicating that it is not RNA-dependent. In addition to its observed physical interaction with Mex67, the lack of MIP6 led to a negative synthetic growth phenotype when combined with mex67-5, a thermosensitive allele of Mex67 28, , at 30°C and at 33°C (Fig 1D). Thus, Mip6 interacts genetically and physically with the general eukaryotic export factor Mex67 and physically with Sus1. Figure 1. Mip6 interacts with components of the mRNA export machinery Sus1-TAP precipitation from WT and ubp8∆ strains. Sus1-TAP and co-precipitating proteins were analyzed on an SDS 4–12% gradient polyacrylamide gel and stained with Coomassie Blue. Bands corresponding to Sus1-TAP and Mip6, identified by mass spectrometry, are indicated. Immunoprecipitation with the TAP antibody using no-tag or Mip6-TAP-expressing cells. Mip6-TAP and Mex67 were detected in INPUT and IP samples by Western blotting. As in (B) in the mip6Δ strain transformed with Mip6-GFP-expressing plasmid with and without RNase A treatment at the indicated concentrations. Growth of WT, and of mip6Δ, mex67-5, and mip6Δmex67-5 mutants. Precultures were diluted in liquid YPD medium. The 10-fold dilutions of cells were then spotted onto YPD plates and incubated for 2 days at the indicated temperatures. Download figure Download PowerPoint The Mex67 UBA domain specifically recognizes Mip6 RRM4 To better characterize the Mip6-Mex67 physical interaction, we used biophysical and structural methods. Pull-down assays showed co-purification of recombinant Mip6 RRM1-4 and Mex67(528–599) (Appendix Fig S1 and Fig EV1A). This Mex67(528–599) construct includes the C-terminal part of Mex67 containing the UBA domain that was found to interact with Mip6 by Y2H 28 (Appendix Fig S1). In contrast, the Mip6 construct lacking the fourth RRM (Mip6 RRM1-3) lost the ability to interact with Mex67(528–599) (Fig EV1A), indicating that the UBA site of Mex67 binds to Mip6 RRM4. This binding was confirmed by size-exclusion chromatography (Fig EV1B). Click here to expand this figure. Figure EV1. Mex67 interacts with Mip6 RRM4 Mip6 RRM4 mediates the interaction of Mip6 with Mex67(528–599). Purified recombinant GST-tagged Mex67(528–599) was bound to pre-equilibrated Glutathione-Agarose beads as indicated. After washing, the beads were incubated with purified untagged recombinant Mip6 RRM1-4 or RRM1-3 as indicated, were washed again, and were then loaded onto a 10% SDS–PAGE gel that was subsequently stained with Coomassie Blue. Stable complex of Mex67(528–599) and Mip6 RRM4(401–480) during gel filtration. Superposition of the chromatogram representing the size-exclusion elution profile of the complex formed between Mex67(528–599) and Mip6 RRM4(401–480), obtained from a 16/60 Superdex 75 gel filtration column at a volume of 67.9 ml, onto the elution profile previously obtained for each of the proteins separately. The elution peak of the complex is shown in blue, and the corresponding fractions visualized by Coomassie staining of a 10% SDS–PAGE gel are marked with a red bar (inset). The light green peak corresponds to the separate elution peak of Mex67(528–599), while the pink peak corresponds to that of the truncated Mip6 RRM4(401–480) construct. Download figure Download PowerPoint Crystallization screening to study the interaction between Mip6 RRM4 and Mex67(528–599) was set up using both proteins in a 1:1 molar ratio. However, only crystals of the Mex67 molecule were obtained. The determined X-ray structure of the obtained Mex67(528–599) crystal (Table EV1, PDB: 6EXZ) contains one molecule of Mex67 in an asymmetric unit that comprises the UBA domain plus the previously unreported presence of 16 N-terminal residues. Mex67(528–599) folding is conserved compared to the previous four-helix bundle solution structure of the Mex67 UBA 33, 34. However, our Mex67(528–599) construct (hereafter named Mex67 N+UBA) revealed the additional presence of two novel short α-helices (helix N1 and N2) (Fig 2A upper panel). This new structure represents a more precise picture of the UBA fold and provides a more complete structural description of the Mex67 C-terminal region than was previously available. Figure 2. The Mex67 C-terminal domain structure and its interaction with Mip6 Ribbon depiction of the crystal structure of Mex67 N+UBA (PDB: 6EXZ). The UBA domain (helices H1–H4) is folded as in previously reported structures (PDB ID: 1OAI). The new N-terminus (shaded light blue) is formed by two short alpha helices (N1 and N2 that fold back into helices H1 and H2). The red bars in the histogram below summarize the analysis of Mip6 RRM4-Mex67 N+UBA binding by NMR titration experiments monitored on the Mex67 N+UBA 1H-15N HSQC spectrum. Peak intensity ratios between free Mex67 N+UBA and a mixture containing Mip6 RRM4 and Mex67 N+UBA (at 15% saturation on Mip6) were plotted against the Mex67 sequence (red bars). The green bars in the histogram are the results of equivalent analysis of the Mip6 RRM4 W442A/Mex67 N+UBA mixture (also at 15% saturation on the Mip6 mutant), and show that W442A abolished the interaction. The signal intensity decreases in the red histogram are mapped on the structure of Mex67 N+UBA. The level of red color saturation directly corresponds to the decrease in signal intensity and reveals the putative Mex67 N+UBA binding site of Mip6 RRM4. Biophysical characterization of the interaction between Mex67(528–599) and Mip6. The upper panel shows the ITC binding isotherms of Mex67(528–599) (N+UBA) titrated into Mip6 RRM1-4(111–480) (green) or Mip6 RRM4(401–480) (red). The lower panel shows the ITC binding isotherms of Mex67 N+UBA titrated into Mip6 RRM4 (red), using the same data as the upper panel, or the Mip6W442A mutant (blue). Immunoprecipitation of the empty plasmid pADH1pr-GFP, Mip6-GFP, or Mip6-ΔRRM4-GFP (left) and of pADH1pr-GFP, Mip6-GFP, or Mip6W442A-GFP mutant (right) expressed from the indicated plasmids in an mip6Δ strain. Mip6-GFP and an empty plasmid were used as positive and negative controls, respectively, in both panels. Mip6-GFP, including its mutant constructions, and Mex67 were detected by Western blotting using the indicated antibodies. The bar graphs at right indicate the quantification of Mex67 IP signal intensity normalized to Mip6. Mean ± SEM from 2 to 5 biological replicates was represented; significant differences from unpaired Student's t-test with Welch's correlations and one-tailed were represented (*P-value < 0.05; ***P-value < 0.001). Growth of WT, Mip6-GFP, and Mip6W442A-GFP strains. Precultures were diluted in liquid YPD medium, and 10-fold dilutions of cells were spotted onto YPD plates. Plates were incubated for 2 days at the indicated temperatures. Download figure Download PowerPoint To quantify the strength of the interaction between the Mex67 N+UBA and Mip6, we performed isothermal titration calorimetry (ITC). ITC showed a similar value between 1 and 2 μM for the apparent dissociation constant (KD) for Mex67 N+UBA and an Mip6 RRM1–4 or an Mip6 RRM4 construct (Fig 2B upper panel). A value of n = 0.96, in agreement with a 1:1 stoichiometry, was obtained for the interaction between Mex67 N+UBA and Mip6 RRM4. Next, we analyzed the Mex67-Mip6 interaction by nuclear magnetic resonance (NMR) to gather structural information about the Mip6 RRM4–Mex67 N+UBA interaction interface. We assigned the NMR spectra of each domain (Appendix Fig S2A and B) and performed titration experiments. However, binding occurred in a chemical exchange regime that caused severe line broadening effects above 15% saturation (Appendix Fig S3); thus, no direct chemical shift mapping could be obtained. However, the same chemical exchange process caused peak intensity losses in the 1H-15N HSQC spectra (Fig 2A red histogram) at low complex saturation (> 15%) that could be mapped on the crystal structure (Fig 2A upper panel, red shading). This mapping suggested that the Mip6 binding interface is at the confluence of helices N1, N2, and H2 of the Mex67 N+UBA. To evaluate the possible involvement of the Mex67 N-terminal extension (helices N1 and N2) in Mip6 recognition, we quantified the energetic contribution by biolayer interferometry (BLI). Experiments using GST-Mex67-UBA and GST-Mex67-N+UBA constructs provided similar KD values (Appendix Fig S4), and no detectable binding was observed with a construct including only the N-terminal extension (GST-Mex67481–544), suggesting that the Mex67 N-terminal extension did not contribute to Mex67 UBA binding and that the changes detected by NMR might be due to subtle conformational rearrangements. In summary, these results show a direct interaction between Mip6 RRM4 and Mex67 UBA domains, likely involving the alpha helix H2. A loop in Mip6 RRM4 including W442 is responsible for Mex67 binding specificity To identify the Mip6 RRM4 structural elements directly involved in Mex67 recognition, we analyzed the effect of titration of Mex67 onto the Mip6 RRM4 1H-15N HSQC spectra. Most of the RRM structural core signals (β–sheet and α-helices) remained unchanged by Mex67 titration, in contrast to variations induced by Mex67 titration that were found in a set of unassigned signals that likely correspond to loop residues (Fig EV2A). Furthermore, of the two tryptophans in the Mip6 RRM4 domain, only W442 was strongly affected by Mex67 binding and therefore was directly involved in the binding (Fig EV2A). This W442 residue is located in an unusually long loop (loop 1) and can be mutated (mip6 RRM4 W442A) without compromising the structural integrity of RRM4, as the mutant NMR spectra were almost identical to those of the wild type (hereafter WT) (Fig EV2B). In contrast to the WT, the NMR spectrum of mip6 RRM4 W442A was insensitive to Mex67 titrations (Fig 2A, green histogram), which confirms that Mip6 W442 is essential for Mip6-Mex67 interaction (Fig EV2C). Additional ITC experiments further demonstrated that this single point mutation in Mip6 (W442A) is critical for Mex67 recognition in vitro (Fig 2B lower panel). Click here to expand this figure. Figure EV2. Mip6 RRM4 NMR analysis Left panel: Superposition of 1H-15N HSQC spectra of the titration of unlabeled Mex67 N+UBA onto the Mip6 RRM4: black (free protein), dark red (50% saturated with unlabeled Mex67 N+UBA), and red (100% saturated). Signals corresponding to the side chain crosspeaks of tryptophans have been marked to show their differential behavior upon Mex67 interaction. Many signals disappear upon titration, most of which were not assigned and likely belong to loops. Right panel: the chemical shift mapping and their representation over the structure of the Mip6 RRM4 model: Shades of blue show non-interacting residues, shades of red show interacting residues, and unassigned residues are shown in gray. Superposition of the 1H-15N HSQC spectra of Mip6 RRM4 (purple) and the Mip6 RRM4 W442A mutant (green). Note the disappearance of the W442 side chain crosspeak in the mutant. The majority of the peaks remain at the same position in the mutant, showing that the structures are very similar. Crosspeaks in the mutant are sharper than those in the WT, showing that it is less prone to aggregation. NMR-monitored experiments of the titration of unlabeled Mip6 RRM4 W442A into 15N-labeled Mex67 N+UBA. There are almost no changes upon the addition of equimolecular amounts of the Mip6 mutant in contrast to the WT case (Appendix Fig S3). Download figure Download PowerPoint To analyze the in vivo relevance of Mip6 RRM4 and its W442 residue in Mip6-Mex67 interaction, Mip6 pull-down experiments were performed using WT cells transformed with plasmids expressing Mip6-GFP, Mip6-ΔRRM4-GFP, or Mip6W442A-GFP. As shown in Fig 2C (left panel), a strong reduction in the level of Mex67 co-purifying with Mip6 was observed for the Mip6-ΔRRM4 mutant compared to WT. Similarly, point mutation of W442A also reduced association of Mip6 with Mex67 to background levels (Fig 2C, right panel). The combined structural and biophysical data show that Mex67 directly interacts with the Mip6 RRM4 through W442 in loop 1, by using the interface of helix 2 (in Mex67). This binding mode can be effectively disrupted in vitro and in vivo by a single point mutation of tryptophan 442 in the Mip6 protein to alanine. Although deletion of MIP6 does not affect normal yeast growth, we created a genomic version of Mip6W442A-GFP using a Mip6-GFP strain to compare the cell growth phenotypes of WT (Mip6-GFP) and mutant Mip6W442A-GFP strains. A dot spot assay showed a growth defect at all tested temperatures for the Mip6W442A-GFP mutant but not for Mip6-GFP (Fig 2D). We concluded that Mip6 W442A mutation, which altered Mip6 interaction with Mex67, affects cellular growth, likely causing a toxic effect. Binding of Mip6 RRM4 to Mex67 N+UBA prevents its association with RNA The above experiments showed that Mip6 RRM4 interacts with the Mex67 UBA. We next tested whether this Mip6 domain could also bind RNA. Polyuridylic acid–agarose bead (poly(U)) pull-downs with different Mip6 constructs showed that Mip6 constructs expressing RRM1/2 or RRM3/4 bound similar to poly(U) (Fig 3A and Appendix Fig S1). The poly(U) binding of Mip6 RRM4 alone was similar to that of Mip6 RRM3/4 (Fig 3A), whereas the binding of Mip6 RRM3 was lower than that of the other constructs (Fig 3A). We tested binding to other RNA homopolymers (i.e., poly(A)) but unfortunately could not extract clear conclusions about protein selectivity. Figure 3. Binding of Mip6 RRM4 to Mex67 N+UBA prevents its association with RNA Purified recombinant Mip6 constructs were bound to pre-equilibrated poly(U) agarose beads. After washing, beads were boiled with SDS sample loading buffer and loaded onto a 10% SDS–PAGE gel that was subsequently stained with Coomassie Blue Input (I), wash (W), and eluate (E), which are shown for each pull-down. BSA was used as a negative control. The asterisk indicates GST from the purification. As in (A) using preincubated complex of Mex67(528–599) with Mip6 RRM4 (left) or Mip6 RRM1/4 construct (right). Mex67(528–599) shows no poly(U) binding when preincubated with Mip6. Download figure Download PowerPoint Because Mip6 RRM4 acts both as a bona fide RNA and as a protein (Mex67 N+UBA) recognition domain, we further investigated crosstalk between these two events. Pull-down experiments showed that, although Mip6 RRM4 and RMM1/4 bind effectively to poly(U) agarose beads (Fig 3A), notably, their complexes of Mip6 RRM4 or Mip6 RRM1/4 with Mex67 N+UBA do not (Fig 3B). Thus, Mip6 RRM4 can bind both a protein Mex67 and poly(U), but apparently not simultaneously in vitro. Mip6 shuttles between the nucleus and the c
更多查看译文
关键词
mip6,mex67 uba domain,mrnas
AI 理解论文
溯源树
样例
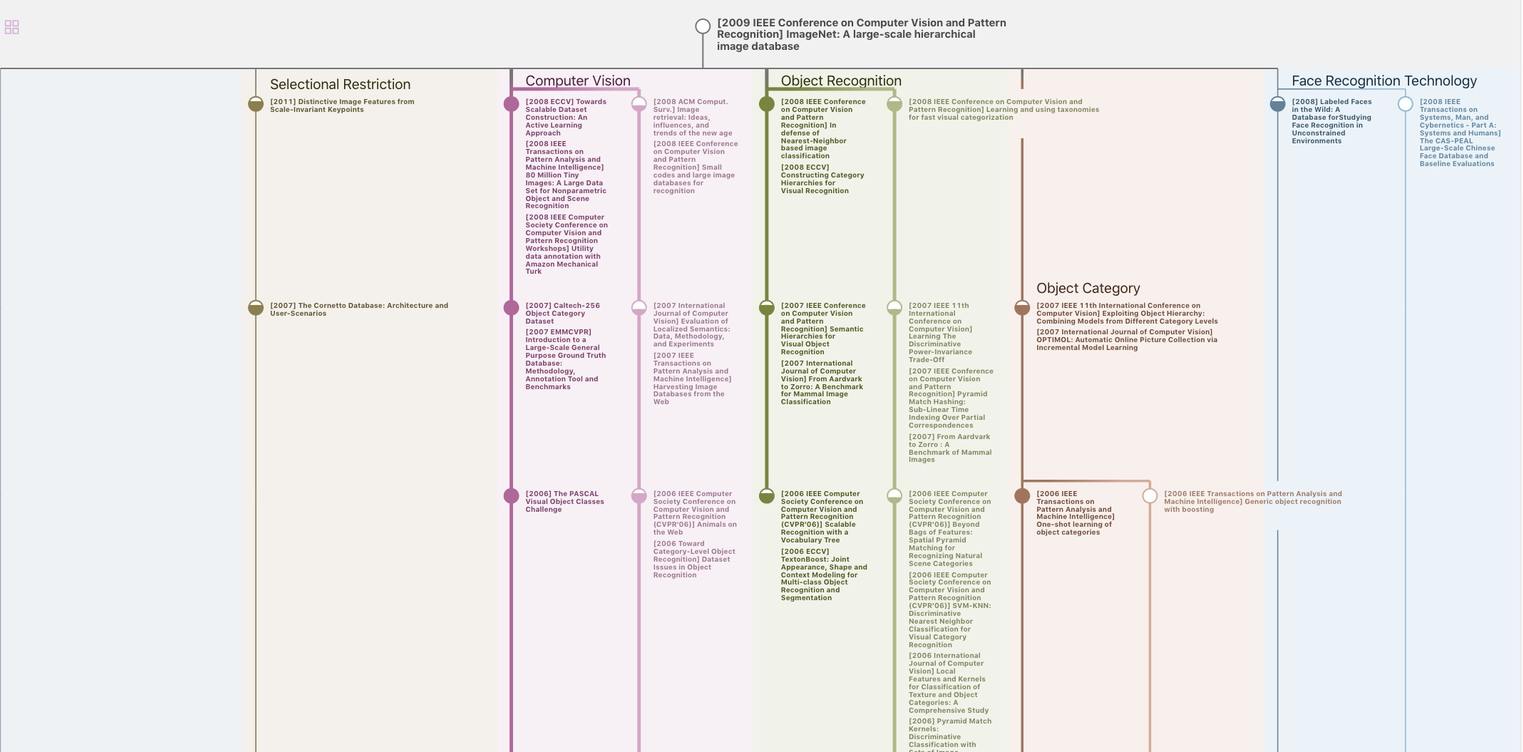
生成溯源树,研究论文发展脉络
Chat Paper
正在生成论文摘要