Author Response: Nuclear Fascin Regulates Cancer Cell Survival
crossref(2022)
Key words
Cancer Progression
AI Read Science
Must-Reading Tree
Example
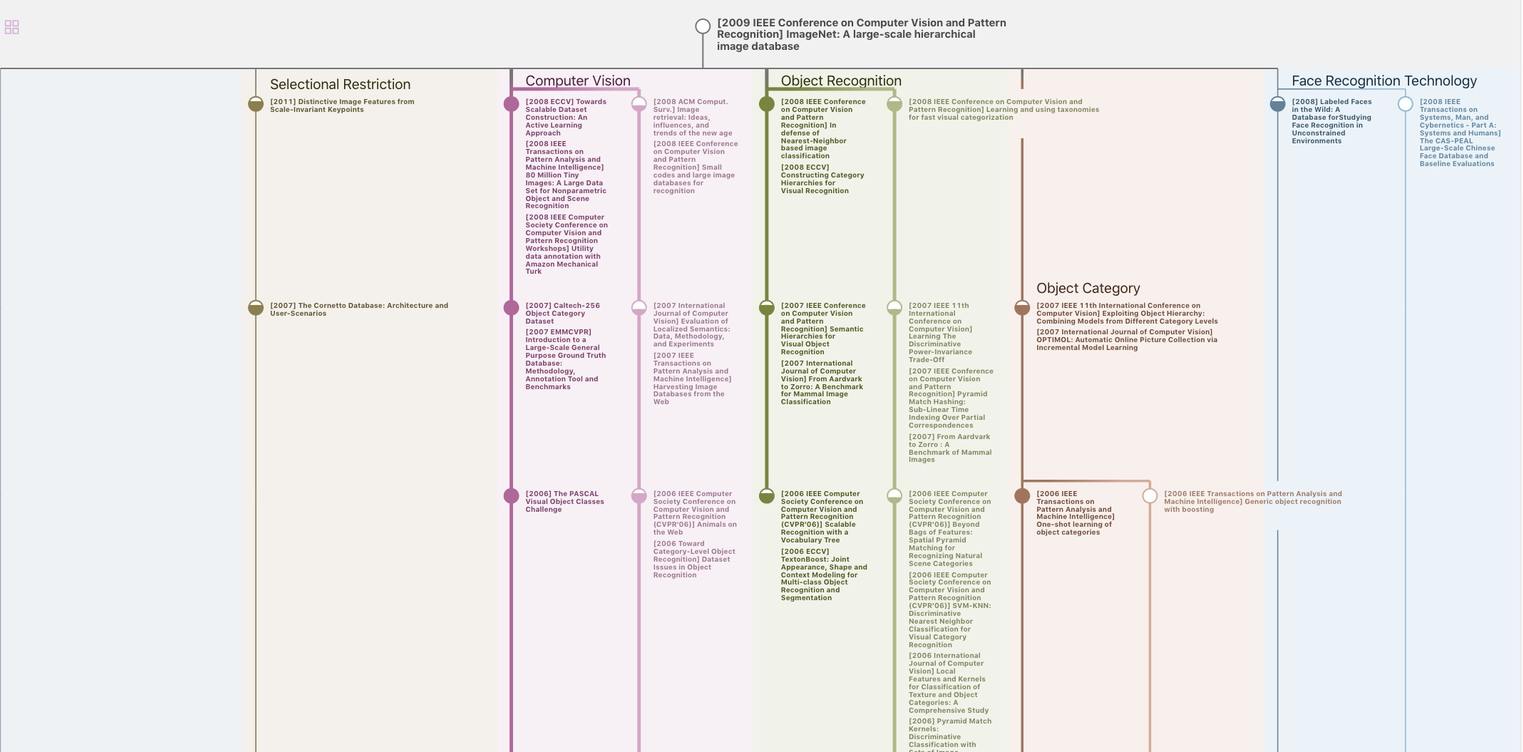
Generate MRT to find the research sequence of this paper
Chat Paper
Summary is being generated by the instructions you defined