Decision Letter: Phasic Oxygen Dynamics Confounds Fast Choline-Sensitive Biosensor Signals in the Brain of Behaving Rodents
crossref(2020)
摘要
Article Figures and data Abstract Introduction Results Discussion Materials and methods Data availability References Decision letter Author response Article and author information Metrics Abstract Cholinergic fast time-scale modulation of cortical physiology is critical for cognition, but direct local measurement of neuromodulators in vivo is challenging. Choline oxidase (ChOx)-based electrochemical biosensors have been used to capture fast cholinergic signals in behaving animals. However, these transients might be biased by local field potential and O2-evoked enzymatic responses. Using a novel Tetrode-based Amperometric ChOx (TACO) sensor, we performed highly sensitive and selective simultaneous measurement of ChOx activity (COA) and O2. In vitro and in vivo experiments, supported by mathematical modeling, revealed that non-steady-state enzyme responses to O2 give rise to phasic COA dynamics. This mechanism accounts for most of COA transients in the hippocampus, including those following locomotion bouts and sharp-wave/ripples. Our results suggest that it is unfeasible to probe phasic cholinergic signals under most behavioral paradigms with current ChOx biosensors. This confound is generalizable to any oxidase-based biosensor, entailing rigorous controls and new biosensor designs. Introduction Acetylcholine (ACh) is an essential modulator of neuronal circuits engaged in high order cognitive operations. During aroused states, high extracellular ACh sets cortico-hippocampal circuits toward memory encoding by enhancing sensory processing, synaptic plasticity and neuronal network rhythmicity (Hasselmo and McGaughy, 2004; Marrosu et al., 1995; Steriade, 2004; Teles-Grilo Ruivo and Mellor, 2013). The latter is particularly relevant in the hippocampus, where ACh plays an important role in the processing of episodic and emotional information via modulation of theta oscillations (Buzsáki, 2002; Gu et al., 2017; Li et al., 2007; Mikulovic et al., 2018; Vandecasteele et al., 2014). Contrarily, low tonic ACh during non-REM sleep permits the occurrence of hippocampal sharp-wave/ripple complexes (SWRs), which are critical for memory consolidation (Buzsáki, 2015; Hasselmo and McGaughy, 2004; Norimoto et al., 2012; Vandecasteele et al., 2014). The current theory on the functional role of ACh in cortical and hippocampal circuits has been mainly derived from brain-state-related correlations of tonic ACh levels with underlying network dynamics, or from strong manipulations of the cholinergic system (Gu et al., 2017; Hasselmo and McGaughy, 2004; Li et al., 2007; Marrosu et al., 1995; Norimoto et al., 2012; Vandecasteele et al., 2014). However, such crude analytic and experimental approaches cannot account for the spontaneous non-stationary interactions between ACh, behavior and neuronal network activity. Recently, fine time-scale measurements of cholinergic activity have provided new insights into this interplay. Fast cholinergic transients in cortical and hippocampal regions have been described in response to sensory sampling, unexpected events, negative reinforcements, and reward-related behavior (Eggermann et al., 2014; Hangya et al., 2015; Howe et al., 2017; Lovett-Barron et al., 2014; Parikh et al., 2007; Teles-Grilo Ruivo et al., 2017). The latter have been captured using electrochemical biosensors in response to detection of cues to rewards and reward approach or retrieval in freely moving rodents (Howe et al., 2017; Parikh et al., 2007; Teles-Grilo Ruivo et al., 2017). The temporally precise alignment of phasic ACh signals to these events hints for a critical role of ACh on the formation of reward-related memories and on the guiding of learned reward-oriented actions. The above-mentioned studies highlight the suitability of enzyme-based electrochemical biosensors to capture phasic release of neurotransmitters and neuromodulators (Chatard et al., 2018). Additionally, amperometric measurements pick-up currents generated by the local field potential (LFP) (Santos et al., 2015; Viggiano et al., 2012; Zhang et al., 2009), making these sensors ideal for studying the interplay between neuromodulatory tone and neuronal network dynamics. The most successful electrochemical ACh-sensing strategy in vivo has relied on the Choline Oxidase (ChOx)-mediated measurement of extracellular choline (Ch), a product of ACh hydrolysis by acetylcholinesterase. The enzyme catalyzes Ch oxidation in the presence of O2, generating H2O2, which is oxidized on the electrode surface (Burmeister et al., 2003; Parikh et al., 2004; Parikh et al., 2007; Teles-Grilo Ruivo et al., 2017; Zhang et al., 2010). However, despite the apparent success of ChOx-biosensors, the factors that can confound their response in vivo at the fast time-scale, such as LFP-related artifacts and O2-evoked enzyme transients, have not been thoroughly addressed. Chemical modification of the electrode surface has been a common approach used to effectively reduce electrodes’ response to electroactive substances (e.g. ascorbate or dopamine) (Burmeister et al., 2003; Zhang et al., 2010; Baker et al., 2015). Cancellation of neurochemical artifacts generating faradaic currents has been further improved in multi-site sensor designs (Burmeister et al., 2003; Santos et al., 2015; Zhang et al., 2010). By differentially coating the recording sites with matrices that contain or lack the enzyme, sites can be rendered Ch-sensitive or not (sentinel sites), enabling differential measurements with improved selectivity. Although the combination of these two strategies has proven effective on neurochemical artifact removal, the differential coating of the recording sites is not optimal to remove capacitive currents arising from fluctuations in the local field potential (Zhang et al., 2009). Although differential measurements are essential to clean biosensor signals (Santos et al., 2015; Zhang et al., 2010), cross-talk caused by H2O2 diffusion from enzyme-coated to sentinel sites poses important constraints on the sensor design. The inter-site spacing required to avoid diffusional cross-talk (typically >150 μm, depending on enzyme loadings) leads to uncontrolled differences in the amplitude and phase of LFP across sites, compromising common-mode rejection. Furthermore, the strategies devised to reduce artifacts that directly generate electrochemical currents (chemical surface modifications or common-mode rejection) and are unrelated to enzymatic response to Ch, do not control for factors influencing immobilized ChOx activity (COA). Given that O2 is a co-substrate of the enzyme, it is crucial to control whether physiological O2 variations can contribute to biosensor responses in vivo leading to distortion of true and detection of false-positive Ch signals. Previous studies have only shown that O2 steady-state responses of ChOx-based biosensors in vitro follow apparent Michaelis-Menten saturation kinetics (Baker et al., 2017; Burmeister et al., 2003; Santos et al., 2015). The relatively narrow linear range of O2-dependent biosensor responses, as compared with estimates of average O2 levels in the brain, has motivated the assumption that ChOx-based biosensors are not affected by in vivo O2 dynamics (Baker et al., 2017; Burmeister et al., 2003). That might, however, oversimplify the effect of O2 on enzymatic activity since its basal levels and activity-related phasic dynamics widely vary across brain regions and experimental conditions (Lyons et al., 2016; Murr et al., 1994; Nair et al., 1987). Furthermore, previous literature has ignored possible non-steady-state (phasic) biosensor responses to O2, which might arise from local consumption and diffusion of enzyme substrates and reaction products in the sensor coating. These putative transient sensor responses to O2 are particularly relevant as they might temporally overlap with fast cholinergic transients. Therefore, the full assessment of biosensors’ O2 dependence requires the characterization of tonic and phasic O2-evoked responses and the simultaneous in vivo measurement of COA (biosensor response) and O2 within the sensor substrate. Yet, when addressed, O2 levels in the tissue have been measured using a separate electrode, often of different geometry and/or having a surface material or modification that differs from the Choline-sensing site (Baker et al., 2015; Dixon et al., 2002; Santos et al., 2015). This approach is therefore prone to bias from heterogeneous tissue O2 dynamics and differential kinetics of electrode responses to O2. Importantly, these studies have only characterized effect of very slow, tonic changes in O2 levels on sensor response. Here, we have implemented a novel sensing approach based on differential modification of recording sites’ electrocatalytic properties toward H2O2, resulting in Ch-sensitive and pseudo-sentinel sites. As Ch (or COA) responses depended solely on the intrinsic properties of the metal surface, we could dramatically reduce the size and increase the spatial density of recording sites by using tetrodes as the electrode support. The Tetrode-based Amperometric ChOx (TACO) sensor provides differential responses to changes in COA, interferents and LFP across four bundled 17 μm diameter Pt/Ir wires. Importantly, this multichannel configuration allows highly sensitive measurement of COA and O2 in the same brain spot by using a tetrode site to directly measure the latter. This has not been possible to achieve with conventional enzyme-based biosensors, whose design was constrained by diffusional cross-talk. We show that the TACO sensor provides a highly selective and sensitive measurement of COA when recording from the brain of behaving animals, effectively suppressing artifacts caused by neurochemicals, LFP and movement. But remarkably, a detailed in vitro characterization and mathematical modeling of biosensors revealed a novel phasic component of sensor’s O2 dependence caused by non-stationary enzyme responses to phasic O2 changes. Accordingly, measurements with the TACO sensor in behaving animals revealed fast temporally- and amplitude-correlated O2 and COA dynamics following locomotion bouts and hippocampal SWRs. Causal analysis of this correlation via local or systemic manipulation of O2 dynamics in vivo demonstrated that O2 transients can cause phasic COA responses. Our results demonstrate that the biosensor’s phasic O2 dependence causes transient biosensor signals in response to physiological fluctuations in O2. The extent and complexity of O2-related confounds is such that extraction of authentic cholinergic dynamics from the signal is not feasible with currently methodology. Importantly, this O2 ChOx-confounding dynamics is associated with behaviorally and physiologically relevant events and warrants important implications for the interpretation of previous studies relying on ChOx and other oxidase-based sensors as well as for the design of future enzyme-based sensors. Results The TACO sensor provides a highly selective differential measurement of COA The TACO sensor is built around Pt/Ir wire tetrode, providing four disc-shaped recording sites with 17 μm diameter in close proximity, resulting in an entire sensor diameter of approximately 60 μm (Figure 1A). Such spatial density of recording sites is ideal for common-mode rejection of LFP-related currents and neurochemical dynamics. At this spatial scale, diffusional crosstalk would preclude the use of sentinel sites by differential coatings, as done in conventional biosensors, including our previous ChOx biosensor design (Burmeister et al., 2003; Santos et al., 2015). Instead, we created pseudo-sentinel and Ch-sensing sites by differentially plating the tetrode wires, modifying their electrocatalytic response toward H2O2. This step was followed by coating the tetrode surface with a common matrix containing ChOx entrapped in chitosan (Santos et al., 2015; Figure 1A). As for the initial plating steps, all tetrode sites were first mildly plated with gold, which marginally increased the electrode surface area, as inferred from impedances at 1 kHz (419 ± 33 kΩ, n = 28 before vs. 370 ± 16 kΩ, n = 44 after gold plating). Despite the slight decrease in impedance, gold-plating significantly decreased the electrocatalysis of H2O2 reduction/oxidation at the metal surface. In enzyme-coated electrodes, gold-plated sites exhibited a nearly fivefold smaller H2O2 sensitivity than an unplated Pt/Ir surface (Figure 1—figure supplement 1). Remarkably, following this first step, gold-plated sites could be rendered H2O2-sensitive upon mild platinization. In enzyme-coated electrodes, there was a nearly 10-fold difference in H2O2 oxidation currents between Au and Au/Pt sites at 0.4–0.6 V vs. Ag/AgCl (Figure 1B). The increase in Au sites’ response to H2O2 above +0.7 V is in agreement with the electrochemical behavior of a pure Au electrode and probably results from the formation of surface oxides (Burke and Nugent, 1997; O'Neill et al., 2004). Interestingly, the response of Au/Pt electrodes to H2O2 was higher than that of unplated electrodes (Figure 1—figure supplement 1), possibly reflecting an increase in the electrode surface area and/or an electrocatalytic effect caused by the deposition of nanostructured platinum over the gold surface (Burke and Buckley, 1996; Domínguez-Domínguez et al., 2008). Even when using common-mode rejection, decreasing the magnitude of interferences in individual sites is desirable. Thus, after plating and coating the tetrode, we electropolymerized m-PD in two of the recording sites, in order to reduce responses to electroactive compounds larger than H2O2 (e.g. ascorbate or dopamine) (Hascup et al., 2013; Santos et al., 2008). The final TACO sensor configuration consisted of all possible combinations of Pt and m-PD modifications of Au-plated recording sites (Figure 1A). Figure 1 with 1 supplement see all Download asset Open asset TACO sensor design and response properties. (A) Schematics depicting the multichannel biosensor design, including the assembly used in in vitro and head-fixed recordings (bottom). HS: head-stage. (B) Voltammogram showing H2O2 sensitivities of gold-plated and platinized sites upon amperometric calibrations at different DC potentials (n = 10). Prior to calibrations, tetrodes were coated with a matrix of chitosan/ChOx. (C) Top shows a representative calibration of a sensor showing the response of different types of sites to step additions of Ch, H2O2, dopamine (DA). and ascorbate (AA). Bottom shows an example of current responses to a sinusoidal 12 mV AC voltage at 0.2 Hz overlaid on top of +0.6 V vs. Ag/AgCl DC voltage. (D) Sensitivities of different sites toward Ch (n = 10 biosensors). Unlike m-PD electropolymerization, platinization significantly increased sensitivity (p<0.0001 and F1,33 = 115 for platinization effect and p=0.87 and F1,33 = 0.03 for m-PD, by two-way ANOVA for unbalanced data). (E) Normalized responses of each tetrode site to Ch, interferent molecules and AC voltage, presented as impedance at 0.2 Hz (n = 5–10). Magnitudes significantly depended on the site modification and on the factor tested (p<0.0001 and F1,113 = 22 for platinization, F1,113 = 109 for m-PD and F3,113 = 12.9 for factors, by three-way ANOVA for unbalanced data). Platinization selectively increased responses to Ch (p<0.0001) while m-PD decreased responses only to DA and AA (p<0.0001). Impedances did not significantly differ across different types of electrode modifications (p>0.99). The rightmost column shows COA signal responses computed from the difference between Au/Pt/m-PD and Au/m-PD sites. Groups were compared by three-way ANOVA followed by Tukey-Kramer post-hoc tests. Data are represented as mean ± CI. The responses of TACO sensors’ sites to Ch, H2O2 and to compounds that can potentially interfere during in vivo measurements were tested by step additions in the beaker at +0.6V vs. Ag/AgCl (Figure 1C, top). In accordance with the voltammograms of H2O2 sensitivities (Figure 1B), the responses of TACO sensors’ gold-plated sites to Ch and H2O2 were much lower than those of platinized sites. On average, like for H2O2, this difference was about 10-fold for Ch, regardless of m-PD electropolymerization (Figure 1D). Contrasting with its lack of effect on Ch sensitivity, m-PD dramatically decreased responses to ascorbate and dopamine, regardless of the site’s metal composition (Figure 1E). In addition, we have also calibrated the impedance of recording sites at low frequency by applying a low-amplitude 0.2 Hz AC voltage on top of the DC offset. This low frequency is of particular relevance, as it overlaps with putative phasic cholinergic dynamics previously reported by us and other groups in anesthetized and freely moving rodents (Howe et al., 2017; Parikh et al., 2007; Santos et al., 2015; Teles-Grilo Ruivo et al., 2017). Impedances calculated from the current oscillations generated by the AC voltage (Figure 1C, bottom) were comparable across all sites (Figure 1E). Collectively, the results summarized in Figure 1E and Table 1 validate the gold-plating approach to produce pseudo-sentinel sites, as it selectively reduces electrode’s response to H2O2. The COA signal most consistently used throughout this study was computed from the differential of m-PD-electropolymerized sites, to exploit the advantages of both differential platings and m-PD electropolymerization, resulting in a high selectivity for Ch (or changes in COA) (Figure 1E and Table 1). As compared to our previous stereotrode design using 50 μm diameter wires, these sensors keep the same Ch response performance, with a limit of detection (LOD) in the low nanomolar range, remarkable for such small electrode surfaces. The TACO sensor response is stable, without significant drop upon in vivo head-fixed recordings (2.45 ± 1.11 pA/μM before and 2.46 ± 0.80 pA/μM after implantation, n = 8, p=0.99, paired t-test), shows high linearity within the physiological range and a T50 response time around 1.5 s (Table 1). Noteworthy, though the response of our sensors is expected to be mostly shaped by Ch diffusion in the coating (Santos et al., 2015), the presented response times are in fact slightly overestimated due to a delay caused by mixing of the analyte in the stirred calibration buffer. Additionally, while m-PD abrogates the response of electropolymerized sites to large electroactive molecules, the differential site modifications provide further information on the signal identity. As the Au site (without m-PD) is more responsive to interferents than to Ch, the neurochemical confounds (NCC) signal (please see Materials and methods section) enables to further infer contaminations by neurochemical confounds in vivo. The differential sites’ responses to different factors can potentially be further exploited by multivariate methods of analysis, and the electrochemical tetrode design employed in TACO sensor can be generalized to other types of sensors in the future. Table 1 Analytical properties of TACO sensors. Individual sites’ analytical propertiesChannel typeCh sensitivity (pA/µM)Ch sensitivity (nA µM−1 cm−2)H2O2 sensitivity (pA/µM)DA sensitivity (pA/µM)AA sensitivity (pA/µM)Impedance (GΩ)Au/Pt/m-PD2.45 ± 0.70 (n = 10)1081 ± 309 (n = 10)2.48 ± 0.93 (n = 8)0.15 ± 0.23(n = 10)0.02 ± 0.013(n = 10)2.90 ± 0.48 (n = 5)Au/Pt2.54 ± 0.64 (n = 8)1118 ± 283 (n = 8)2.86 ± 1.10 (n = 6)7.48 ± 1.86(n = 8)0.22 ± 0.20(n = 8)3.38 ± 0.56 (n = 5)Au/m-PD0.27 ± 0.12 (n = 10)118.2 ± 53 (n = 10)0.22 ± 0.16 (n = 8)0.13 ± 0.23 (n = 10)0.007 ± 0.004 (n = 10)3.11 ± 0.77 (n = 5)Au0.25 ± 0.086 (n = 9)111.5 ± 38 (n = 10)0.29 ± 0.08 (n = 7)8.24 ± 1.84 (n = 9)0.19 ± 0.16 (n = 9)3.65 ± 0.59 (n = 5) Analytical properties for COA measurement (Au/Pt/m-PD - Au/m-PD)Ch sensitivity (pA/µM)Limit of detection (nM)Linearity, [Ch]<30 µM (R2)Response time (s)DA sensitivity (pA/µM), selectivity ratioAA sensitivity (pA/µM), selectivity ratio2.18 ± 0.73 (n = 10)28 ± 0.011 (n = 10)0.9996 ± 0.0003 (n = 10)1.4 ± 0.4 (n = 10)0.022 ± 0.38 (n = 10), 101:10.013 ± 0.012 (n = 10), 169:1 The data are given as the mean ± CI (95%). The number of sensors tested is given in parentheses. Data were collected from calibrations on the day after m-PD electropolymerization. Freely moving recordings validate the TACO sensor suppression of current-generating artifacts and suggest a potential O2 modulation of COA in vivo In order to test whether the TACO sensor can measure fast COA transients devoid of artifacts that directly generate electrode currents in behaving animals (regardless of a possibly underlying O2 modulation of COA), we have first performed recordings in freely moving animals. We simultaneously recoded from the CA1 pyramidal layer using the TACO sensor and LFP across hippocampal depth using a 32-channel linear silicon probe implanted in the proximity of the biosensor (Figure 2A). Extracellular electrophysiology allowed us to directly validate the amperometric measurement of high-frequency LFP (Figure 2—figure supplement 1). The COA signal was cleaned by subtraction of the m-PD-electropolymerized pseudo-sentinel from the Ch-sensing sites’ signal, upon frequency-domain correction of the pseudo-sentinel amplitude and phase (please see Materials and methods) (Santos et al., 2015). This procedure led to substantial removal of fast current fluctuations ascribed to LFP (example recording in Figure 2B, top). Accordingly, the median spectral power at ~1–20 Hz of the signal derived from the Au/Pt/m-PD site during both NREM sleep and wake periods decreased more than two orders of magnitude after the signal cleaning procedure (Figure 2B, bottom). The cleaned COA signal tonically changed across different brain states reaching higher values during active wakefulness and REM sleep than during NREM sleep (Figure 2—figure supplement 2). This dynamics is compatible with expected brain state-dependent ACh changes (Hasselmo and McGaughy, 2004; Marrosu et al., 1995), but remarkably, COA also fluctuated on the time-scale of seconds within brain states. Figure 2 with 2 supplements see all Download asset Open asset TACO sensor provides a highly sensitive and selective measurement of COA in freely behaving animals. (A) Left panel shows a simplified diagram of the electronic circuits in freely moving recordings. The amperometric measurement (one channel for simplicity) was based on a voltage clamp circuit. Rf: feedback resistor (1 GOhm); A1, A2: OP amps; Vcmd: command voltage; Im: measured current (acquired as analog voltage signal). Vcmd is set on the MHS-BR4-VA box and sent to the head-stage. Its output is acquired by an Open-Ephys system. Electrophysiological setup is depicted in bottom. Vm: measured voltage output. The amplified LFP signal is acquired by the Neuralynx system. Right panel depicts the arrangement of a TACO sensor and a 32-channel silicon probe chronically implanted in the hippocampus of a rat. Both probes were attached to microdrives. (B) Top, segment of NREM sleep recording, a raw signal (low-pass filtered at 1 Hz), cleaned COA and confound components. Bottom, spectrum of the raw and clean COA signal during wake (n = 10) and NREM sleep periods (n = 19). Data shown as medians ± CI. (C) Average low frequency (1 Hz low-pass filtered) biosensor signals and high frequency power spectrograms triggered to SWRs detected from a silicon probe channel in CA1 pyramidal layer (top quartile of all ripples sorted by power, n = 2019). Average raw (top), cleaned (middle) COA responses and LFP spectrogram triggered on SWRs (bottom). (D) Average raw (top) cleaned COA responses (middle), average speed (middle) and LFP spectrogram (bottom) triggered to peaks in rat speed in an open-field arena (n = 127). Data are represented as mean ± CI, except in B. Hippocampal SWRs and arousal-related locomotion bouts are discrete events associated with major phasic changes in hippocampal network activity, occurring during sleep or wakefulness respectively (Buzsáki, 2002; Buzsáki, 2015; Sirota et al., 2003). Thus, we tested whether these events could correlate with phasic COA dynamics. Hippocampal SWRs were detected from a silicon probe site in the CA1 pyramidal layer during NREM sleep. Average raw biosensor signals triggered on the peak of SWRs showed a prominent peak in all TACO sensor’s sites (Figure 2C, top). The similarity of peak amplitudes suggests an LFP-related origin of these currents, which was virtually absent in the cleaned signals (Figure, 2C, middle), and reflects the slow dynamics of the sharp wave. The high magnitude of this LFP artifact emphasizes the importance of the common-mode rejection approach in revealing COA dynamics that would otherwise be masked. Interestingly, regardless of m-PD electropolymerization, the cleaned COA signal showed a peak lagging the SWR by ~3 s. Importantly, the slow and small amplitude dip in the neurochemical confounds signal excludes the role of interferents (e.g. ascorbate or a monoamine) in the COA transient (Figure 2C, middle). Bouts in locomotion, detected as peaks in the rat running speed, were associated with transient increases in theta power (Figure 2D, bottom) and with a transient current deflection in all TACO sensor sites (Figure 2D, top). Slow time scale currents that might have neuronal, muscle or movement artifact origin were cleaned, similar to SWRs, revealing a slow peak in COA signals following locomotion bouts, but not in the neurochemical confound signal assuring negligible contribution of these interferences in the COA signal (Figure 2D middle). These results highlight the usefulness of our multichannel pseudo-sentinel approach to discriminate between authentic changes in COA and different sorts of current-inducing interferents, including LFP- and movement-related artifacts and neurochemical dynamics. Nevertheless, it is noteworthy that phasic changes in COA were associated with the most salient sources of non-stationarity in hippocampus, namely arousal/locomotion and SWRs (Buzsáki, 2002; Buzsáki, 2015; Sirota et al., 2003). These phenomena can potentially correlate with changes in both ChOx substrates, Ch (Norimoto et al., 2012; Vandecasteele et al., 2014; Hasselmo and McGaughy, 2004; Marrosu et al., 1995; Reimer et al., 2016; Teles-Grilo Ruivo and Mellor, 2013) and O2 (Zhang et al., 2019; Ramirez-Villegas et al., 2015), leading to transient modulation of COA. Thus, our freely moving results further emphasized the need for a detailed investigation of the O2 effect on the COA signal. Biosensor’s COA responses to oxygen have tonic and phasic components Since O2 is a co-substrate of oxidases, physiological O2 variations potentially affect the response of oxidase-based biosensors. However, this issue has not been thoroughly addressed in previous studies (Baker et al., 2015; Chatard et al., 2018; Dixon et al., 2002; McMahon et al., 2007; Santos et al., 2015). Here, we sought a detailed investigation of the biosensor O2-dependence in vitro, enabling the assessment of O2 effect on COA at multiple time scales (i.e. tonic and phasic dynamics). In these experiments, we took advantage of the TACO sensor configuration to sample COA and O2 dynamics from the same spot, necessary for accurate evaluation of O2-dependence of the COA signal. These recordings were performed using a new customized head-stage allowing independent control of the potential applied on each recording site. Oxygen measurement was achieved at a negative potential by O2 reduction on a gold-plated site, which was typically maximal at −0.4 V vs. Ag/AgCl (Figure 3A). In these experiments, clean COA and O2 signals were obtained by subtraction of Au/Pt/m-PD (at +0.6 V) and Au (at −0.2 V) by the pseudo-sentinel m-PD site, respectively. Figure 3 with 2 supplements see all Download asset Open asset Biosensors generate tonic and phasic COA components in response to O2. (A) The voltammogram shows the DC voltage-related sensitivity of gold-plated sites toward H2O2 (n = 10) and O2 (n = 5). (B) An example of an in vitro O2 calibration. Upon removal of O2 from PBS containing 5 μM Ch, 5 μM O2 additions (arrows) were performed until the tonic sensor response saturated. (C) Representative cumulative tonic and phasic responses as a function of O2 baseline after each addition. Tonic data were fitted with Michaelis-Menten equation, resulting in a KmO2app of 0.97 μM (CI = 0.818–1.126 μM) and Imax = 13.2 pA (CI = 13.04–13.37 pA), with RMSE = 0.08 pA. Phasic responses were fitted to the Hill equation, yielding K0.5O2 = 8.6 μM (CI = 7.58–9.62 μM), Hill coefficient n = 2.31 (CI = 1.74–2.88) and Imax = 22.4 pA (CI = 20.6–24.1), with rmse = 0.50 pA. (D) K0.5O2app values of tonic and phasic components from all biosensors (n = 14). Averages and error bars are medians and CIs. Groups were significantly different (sign test, p<0.0005). (E) T90 rise times of O2 steps and COA peaks following O2 additions (n = 72–95). Bars and error bars represent medians and 95% CIs, groups were significantly different (p<0.0001, Wilcoxon rank sum test). (F) Biosensor efficiency (Ch/H2O2 sensitivity ratio) as a function of KmO2app (n = 6). For illustrative purposes, data were fitted with an exponential function. Spearman correlation between variables was −0.9 (p=0.028). (G) Maximal phasic response to O2 (from each biosensor calibration) as a function of KmO2app (n = 14, rspearman = −0.86, p<0.0001). Data were fitted with an exponential decay curve (decay constant k = 0.4 μM−1, CI = 0.031–0.78 μM−1, rmse = 0.68 μM). (H) Amplitudes of phasic ChOx responses divided into low and high-KmO2app groups (n = 7 per group) following O2 additions. Control phasic O2 using the same algorithm is plotted for the same groups (n = 6 for high-KmO2app and n = 4 for low-KmO2app). Low-KmO2app COA transients were h
更多查看译文
关键词
Metabolic Regulation
AI 理解论文
溯源树
样例
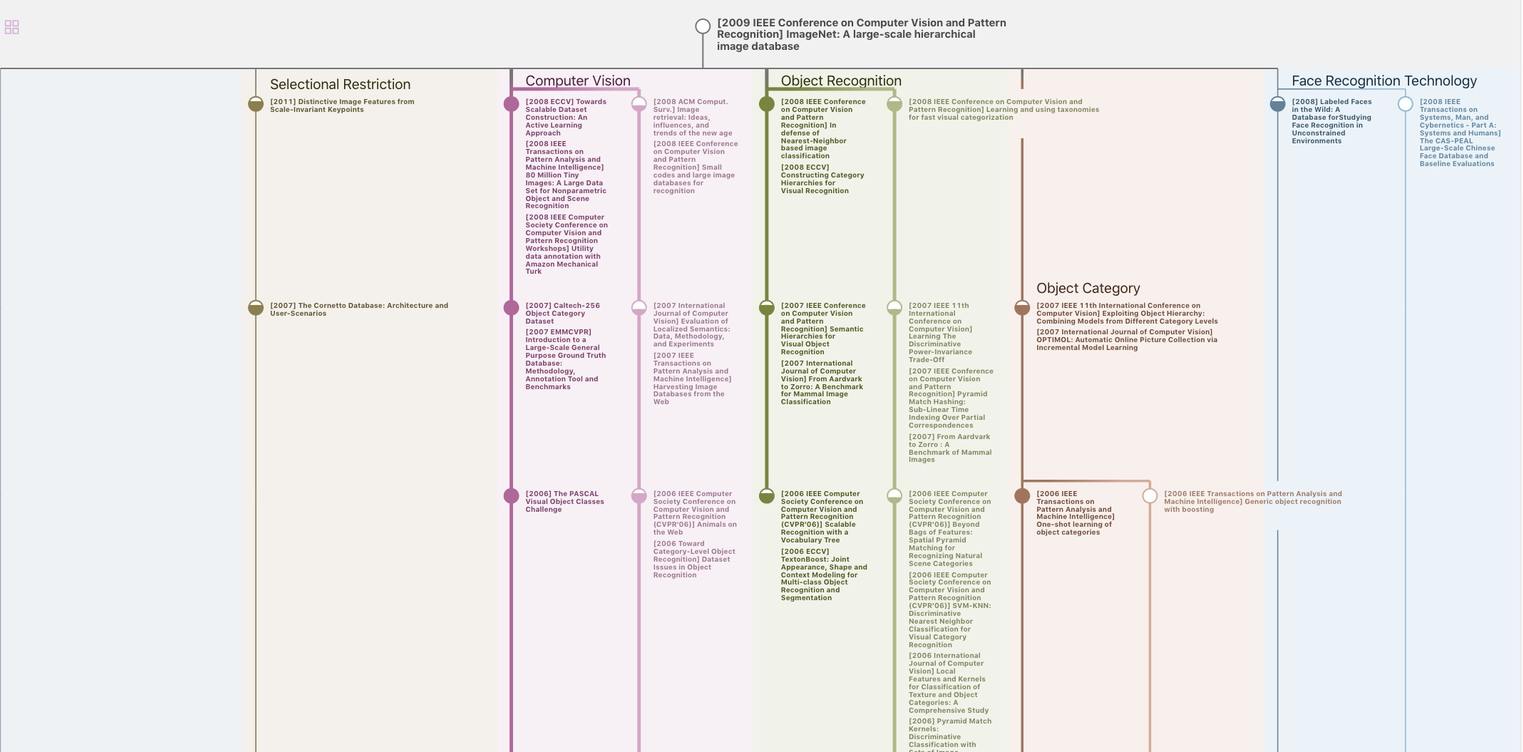
生成溯源树,研究论文发展脉络
Chat Paper
正在生成论文摘要