Immobilization of Trametes hirsuta laccase into poly(3,4-ethylenedioxythiophene) and polyaniline polymer-matrices
Journal of Power Sources(2011)
摘要
Research highlights ► In situ entrapment of Th L in PEDOT film to catalyze the oxygen reduction. ► RVC foam-based biocathode: current density of 1 mA cm −3 . ► RVC foam-based biocathode: pH sensitive with an optimum at pH 4.2. ► Laccase activated polymerization of aniline to immobilize Th L in biocathode. Abstract The immobilization of Trametes hirsuta laccase ( Th L) in the poly(3,4-ethylenedioxythiophene) (PEDOT) and polyaniline (PANI) matrices was carried out in order to study the catalytic effect of Th L in different biocathode structures in a biofuel cell application. By using 2,2′-azinobis (3-ethylbenzothiazoline-6-sulfonate) (ABTS) as a mediator compound, the immobilized Th L in both polymer matrices, exhibited catalytic activity for the reduction of oxygen into water. The amount of Th L was adjustable in the PEDOT matrix by controlling the working parameters, such as the charge density used in the electropolymerization of EDOT monomer and the Th L concentration used in the electropolymerization electrolyte. In the PEDOT biocathode structure, the utilization of porous material as the PEDOT supporting template was studied in order to improve the current density generated per unit area/volume. Reticulated vitreous carbon foam (RVC foam) was chosen as the PEDOT supporting template material and the biocathodes were manufactured by in situ entrapment of Th L into PEDOT films polymerized on the RVC foam. These biocathodes possessed a high cathodic open circuit potential and produced a large current density, reaching 1 mA cm −3 at 0.45 V when 19.5 μg ml −1 of Th L was used in the electrolyte. The performance of these biocathodes was extremely sensitive to variations in pH and the optimal working pH was around 4.2. The biocathode reserved 80%, 50%, and 30% of the catalytic activity after storage in a +4 °C buffer solution for 1 day, 1 week, and 1 month, respectively. The PANI matrix was prepared in a form of printable ink where Th L was in situ entrapped in the PANI matrix during the laccase activated polymerization of aniline using a chemical batch reactor method. Different amounts of the Th L-containing printable PANI ink were then applied on carbon paper and the performance of the ink was subsequently electrochemically characterized. In this way, not only two different polymer matrices, but also two different matrix manufacturing procedures could be compared. Keywords Enzymatic biofuel cell Laccase Biocathode Polyaniline Poly(3,4-ethylenedioxy-thiophene) Reticulated vitreous carbon foam 1 Introduction Biological fuel cells (biofuel cells), are devices using enzymes as catalysts to convert chemical energy to electrical energy. These devices have been recognized as a new type of energy conversion technology and they have attracted considerable attention in the past two decades [1–3] . Due to the green way of producing energy, biofuel cells are ideal power sources for low power-demanding electronic devices, such as, radio frequency identification tags, and miniaturized implantable devices, like pacemakers and glucose sensors for diabetics. A low current output is a general problem in the present biofuel cell designs, and hence the improvement of the current output of the cell is a primary task when developing biofuel cells into a mature technology for applications. One solution to this problem is an efficient enzyme immobilization method as a means to increase the enzyme loading per unit area/volume of the matrix. To date, several different strategies for the immobilization of the enzyme in different biocathode/bioanode constructions have been developed. The most popular immobilization methods include: ‘wired’ enzyme immobilization in redox polymer hydrogels [4–6] , enzyme immobilization in sol–gel derived silica materials [7–9] , enzyme immobilization incorporated with carbon nanotubes [10–12] , and enzyme immobilization in conducting polymers [13] . The different enzyme immobilization methods have particulars for the method specific benefits. The immobilization of enzyme in meso-porous silica materials, for example, can facilitate the retaining of the enzyme activity after the immobilization. The unique properties of the carbon nanotubes, on the other hand, offer the possibility to promote the electron transfer in a way that mimics ‘electrical wires’. Further, enzyme immobilization in conducting polymers, allows for electrochemical deposition of films with controlled thickness and ease of manufacturing on a specific substrate, which offers many possibilities to incorporate diversified materials with favorable features into the electrode construction. The enzyme immobilization in conducting polymers was originally applied in the construction of biosensors [14,15] . Polyaniline, polythiophene and especially polypyrrole and its amphiphilic derivatives have been widely used as matrices for enzyme immobilization in different biosensor constructions. The enzyme can be immobilized into these polymer films by physical entrapment or covalent attachment [16] . Furthermore, conducting polymers have also exhibited electrochemical wiring ability in the electron transfer between the enzyme and the current collector for some particular enzyme cases, such as lactate dehydrogenase immobilization in polyaniline–polyanion composite films [17] and quinohemoprotein alcohol dehydrogenase immobilization in polypyrrole films [18] . It is well known, that there is significant overlap in the working principles of biosensors and biofuel cells and the development of biosensors has therefore provided a substantial technological input for the current biofuel cell development [1] . Enzyme immobilization into conducting polymers has, thus, also been applied in the biofuel cell cathode/anode constructions [13,19] . When biofuel cells are considered, one of the most important tasks is to try to increase the modest current output of the present biofuel cell designs. This calls for the maximization of the enzyme loading per unit area/volume of the matrix as well the enhancement of the immobilization efficiency. Here, two different polymer matrices with different polymerization methods have been studied, as candidates for the construction of a biofuel cell cathode. The first alternative was to immobilize enzyme into conducting polymer films generated on a 3-dimensional porous reticulated vitreous carbon (RVC) foam to maximize the enzyme loading on unit volume. RVC foam is a material with a high void volume, composed solely of vitreous carbon. It has been used as porous electrode material in electrochemical applications, which require very high current distribution areas and low electrical and fluid flow resistance [20] . RVC foam was therefore chosen as the first template candidate in the construction of a biofuel cell cathode. Further, poly(3,4-ethylenedioxythiophene) (PEDOT), was chosen as the first enzyme immobilization matrix due to the interesting properties of PEDOT films, such as high electrical conductivity and excellent inherent environmental stability [21,22] . Hence, RVC foam was used as the conductive substrate for the electrochemical deposition of a PEDOT film, to increase the enzyme loading per unit volume and facilitate the oxygen transport in the cathode side. In our previous work [23] , the electropolymerization of PEDOT films on different carbon based paper and foam materials was systematically studied, providing valuable information on the incorporation of RVC foam and PEDOT based materials into the biocathode construction. Laccase (EC 1.10.3.2) is an oxidoreductase containing four copper ions, which is a member of the blue oxidase family [24] . Laccase catalyzes the oxidation of the substrate, which is an electron donor, with the concomitant reduction of molecular oxygen into water. The catalytic sites of laccase consist of four copper ions, which can be classified in accordance to their spectroscopic characteristics as the T1, T2 and T3 sites. The T2 and T3 copper ions form a trinuclear cluster site, which is responsible for the oxygen binding and the reduction of oxygen into water. The function of T1 site involves electron abstraction from electron donors with a subsequent intramolecular electron transfer via a His-Cys-His bridge to the T2–T3 copper cluster. In the cathodic construction of biofuel cells, laccase has been typically employed as the catalyst in combination with 2,2′-azinobis (3-ethylbenzothiazoline-6-sulfonate) (ABTS) as mediator. The role of the mediator is to shuttle electrons from the current collector to the active centers of laccase [19,25,26] . The enzymatic and physico-chemical properties of laccase depend dramatically on the origin of the enzyme. Trametes hirsuta laccase used in our research was produced from the white rot basidiomycete T. hirsuta , abbreviated as Th L. It has a comparatively high redox potential of the T1 center, 0.78 V vs NHE and, hence, its employment in the biofuel cell cathode construction, results in a high cathodic onset for the open circuit potential of the cell [27] . In the present work, the immobilization of Th L into PEDOT films was achieved through in situ entrapment during the electropolymerization of EDOT on the conductive template. The in situ entrapment of Th L was performed by the incorporation of negatively charged Th L molecules into the positively charged oxidized form of the conducting polymer. Biocathodes were manufactured through the entrapment of Th L into PEDOT films generated on RVC foam. These biocathodes were further optimized by tuning the conditional parameters, such as film growth rate, film thickness, and Th L concentration. Polyaniline (PANI) based ink was chosen as the second matrix material for the Th L immobilization, as Th L can be in situ entrapped in the PANI matrix during the laccase activated polymerization of aniline using a chemical batch reactor method. The capability of laccase on catalyzing the chemical synthesis of water soluble, conducting polyaniline has been reported [28,29] . This enzymatic approach is an environmental friendly alternative route for the synthesis of PANI, which is applied under much milder working conditions, than in the traditional chemical polymerization using high concentrations of strong acids. To construct the enzyme electrode, a PANI ink made from the laccase-catalyzed polymerization was developed as the matrix for the Th L immobilization. The predominate advantages of the Th L-containing PANI ink are the printability of this based conducting ink, easy scalability, and mass processability on paper or cardboard based template materials. Furthermore, the performance of both Th L immobilization matrices towards the reduction of oxygen into water was evaluated using electrochemical characterization methods. The pH dependency of the PEDOT based biocathode was also studied in order to find out its working pH profile. Further, the storage stability of the PEDOT based biocathode was examined for the application in biofuel cells. 2 Experimental 2.1 Materials Th L was provided by VTT Technical Research Centre of Finland as a stock enzyme solution in 20 mM pH 5.0 citrate buffer. Th L produced in its native host was purified in two chromatographic steps as described in the literature [30] . The enzyme activity of this purified protein preparation was 421 U ml −1 (on ABTS at pH 4.5, 25 °C) and the protein concentration was 3.9 mg ml −1 , measured with Bio-Rad DC protein assay kit. The monomer, 3,4-ethylenedioxythiopene (EDOT, >97%), was obtained from Bayer AG. 2,2′-Azinobis (3-ethylbenzothiazoline-6-sulfonate) (ABTS) and potassium nitrate were obtained from Sigma–Aldrich. All the other chemicals used to prepare the buffer solutions were of analytical grade. RVC foam with 100 pores in. −1 , abbreviated as RVC 100ppi, was purchased from ERG Materials and Aerospace Corporation (USA). Slices of RVC 100ppi, with a width of 9 mm and a thickness of 2 mm, were cut from the original piece of RVC 100ppi and were used as working electrodes in the electropolymerization. Materials used in enzymatic polymerization of aniline were aniline (BASF), ABTS (Sigma–Aldrich), polyvinyl alcohol grades Mowiol 8-88 and Mowiol 40-88 (Kuraray), citrate buffer solution pH 4.5, laccase from fungus T. hirsuta ( Th L from VTT, specific activity 732 U mg −1 ) and multi walled carbon nanotubes (Showa Denka). Carbon paper GDL35 AA (gas diffusion layer), with a thickness of 300 μm, was purchased from SGL Carbon Group (Germany). Water was purified with Milli-Q ® apparatus to a specific resistivity of 18.2 MΩ cm, which was used to prepare all the solutions. 2.2 Methods 2.2.1 Manufacture of biocathode Glassy carbon (GC) electrode (projected area 0.07 cm 2 ) was polished with 0.3 μm and 0.05 μm aluminium oxide powder to a mirror-like finish and subsequently rinsed with water and ethanol and cleaned in an ultrasonic bath for at least 5 min before use. Biocathodes were manufactured on the first matrix material (RVC foam) through in situ entrapment of Th L into PEDOT films during the electropolymerization. The electropolymerization was performed in a one-compartment, three-electrode cell with a GC electrode ( d = 3 mm) or a slice of RVC 100ppi as the working electrode, a glassy carbon rod as the auxiliary electrode and with a Ag/AgCl/KCl (saturated) electrode as the reference electrode. A 0.05 M pH 6.0 succinate buffer solution, containing 0.01 M EDOT, 0.05 M KNO 3 , and Th L with different concentrations (3.9, 7.8, and 19.5 μg ml −1 ), was typically employed as the electrolyte for the electropolymerization. The electrolyte was thoroughly purged with N 2 for 15 min before electropolymerization and N 2 supply was kept to blanket the electrolyte during the electropolymerization. PEDOT films with Th L entrapped in the polymer films as dopant, were generated on the corresponding working electrodes under a constant potential of 0.915 V. The passed charge was varied by controlling the duration time of the electropolymerization. In situ entrapment of Th L during the laccase activated polymerization of aniline using chemical batch reaction method and simultaneously preparing a printable ink for cathode construction was made in the following way. In the first stage the binder mixture was prepared using a mixture of succinate buffer solution with pH 4.5 (15 ml) and polyvinyl alcohol Mowiol 40-88 and Mowiol 8-88 in 10% dry material content in succinate buffer. The amount of polyvinyl alcohol was 4% of the mixture and it was used to enhance the adhesion to the printed surface. 2% of carbon nanotubes were added and mixed over night with magnetic stirrer and 8 min with ultrasonic treatment. Carbon nanotubes were used to enhance the ink porosity and to improve the conductivity of the ink. 0.568 g of ABTS was dissolved in the mixture and 125 μl of aniline was added. Mixing was continued in ice-bath for 1 h. Th L was added in amounts of 102 U (PANI ink 1) and 138 U (PANI ink 2). Mixing was continued for overnight and the temperature was let to rise slowly to room temperature. The final product was a black coloured, slightly greenish ink like dispersion. This Th L containing PANI ink was, further, applied on a disc of carbon paper GDL35 AA with a diameter of 5 mm. For the electrochemical evaluations, the GDL35 AA disc with PANI ink applied was then inserted in an electrode structure, where the surface facing the electrolyte solution was covered with a doubly folded cellophane membrane. 2.2.2 Electrochemical measurements Both cyclic voltammetric and chronoamperometric measurements were performed using an IviumStat potentiostat (the Netherlands) in a conventional three-electrode cell equipped with an Ag/AgCl/KCl (saturated) reference electrode and a glassy carbon rod as the auxiliary electrode. The working electrodes were the PEDOT film based biocathodes generated on a GC electrode or RVC 100ppi foam and the PANI ink based biocathodes drop-cast on a carbon paper material and sealed within the electrode structure with the double layered cellophane membrane. The working electrolyte in all cases was 0.05 M pH 4.5 succinate buffer containing 0.2 mM ABTS. The electrolyte was purged with O 2 for half an hour before performing each measurement, and the O 2 supply was kept to blanket the electrolyte during the entire measurement. Polarization curves ( j – E ) for the different biocathodes were plotted by extracting the steady-state current from the chronoamperometric measurements. To investigate the pH dependence of Th L immobilized in PEDOT films, the chronoamperometric measurements of the biocathodes were performed under 0.45 V in a conventional McIlvaine buffer containing 0.2 mM ABTS (pH 4–7). To evaluate the storage stability of the biocathode with Th L immobilized in the PEDOT film, chronoamperometric measurements of the biocathode were performed under 0.45 V after different storage times. The biocathode was kept in a pH 4.5, 0.05 M succinate buffer in +4 °C during the storage. 2.2.3 Scanning electron microscopy The morphology of the PEDOT film with Th L entrapped in the PEDOT film structure, generated on RVC 100ppi, was imaged using a scanning electron microscope, SEM model LEO 1530 (LEO Electron Microscopy Ltd., Germany). The sample was mounted with a conductive tape to a metal plate for direct observation without any coatings. 3 Results and discussion 3.1 In situ entrapment of Th L into a PEDOT film during electropolymerization Prior to manufacturing the biocathode using RVC foam as electrode material, the immobilization of Th L into PEDOT films was performed on the GC electrode due to its well-defined surface area. Since both GC electrode and RVC foam are made of vitreous carbon, no difference related to the nature of the electrode materials on the enzyme immobilization is foreseen in these two cases. For electropolymerization, a pH 6 buffer solution was employed as electrolyte to keep Th L negatively charged (the PI value of Th L is around pH 4.7) and 0.05 M KNO 3 was added as the supporting electrolyte to increase the ionic conductivity of the electrolyte, since comparatively low concentrations of Th L were typically used. However, to avoid the total replacement of Th L to nitrate as counterions in the PEDOT film, high concentrations of KNO 3 were avoided. Furthermore, in the potentiostatic electropolymerization of PEDOT, a low electropolymerization potential, 0.915 V, was used to make a compromise between the growth of the polymer and the diffusion of Th L into the polymer network. PEDOT films with Th L entrapped were examined by cyclic voltammetric measurements to clarify whether the immobilized Th L reserved the catalytic activity towards the reduction of oxygen. As seen in Fig. 1 a, a reversible redox-wave at E 1/2 = 0.52 V, corresponding to the redox couple ABTS 2− /ABTS − , appeared in all three voltammograms. The redox potential of this couple is quite close to that of Th L T1 Cu + (0.78 V vs NHE). Therefore, the combination of Th L and ABTS, as the catalyst and the mediator, respectively, offers the benefit of only a small potential loss on the cathode side. A PEDOT film, generated on the GC electrode by using 1 mC charge in the electropolymerization, demonstrated much larger redox peaks of the ABTS 2− /ABTS − couple compared with the bare GC electrode, due to the increased active surface area of the electrode. This can be seen in Fig. 1 a, curve 2. When Th L was present in the electropolymerization electrolyte, an even larger cathodic current was obtained in the potential range 0–0.4 V, compared with the plain PEDOT film, as seen in Fig. 1 a, curve 3. It was, hence, evident that Th L was entrapped from the electrolyte into the PEDOT film preserving its catalytic activity to the reduction of oxygen. Since Th L was entrapped as dopant into the polymer film, the amount of entrapped Th L was dependent on the amount of PEDOT polymer generated on the support. This was verified by cyclic voltammograms of PEDOT films with entrapped Th L, generated by using variant amount of charge in the electropolymerization, as shown in Fig. 1 b. The working parameters for obtaining the curves 1, 2 and 3 in Fig. 1 b were identical except that the amount of charge used in the electropolymerization was varied by controlling the duration time. A larger cathodic current was obtained with increasing the amount of charge used in the electropolymerization. This indicates that more Th L was entrapped into the PEDOT films when more polymer was produced as the result of the prolongation of the electropolymerization time. These films also demonstrated larger catalytic capacities on the reduction of oxygen. Until now, it has been shown that Th L entrapped into PEDOT films retains its catalytic activity towards the oxygen reduction into water and the entrapped amount of Th L is controllable by adjusting the experimental parameters. It is, thus, concluded that in situ entrapment of Th L into PEDOT films is applicable for the purpose of immobilizing the biocatalyst in the construction of the cathode of biofuel cells. 3.2 Biocathodes manufactured by in situ entrapment of Th L into PEDOT films generated on RVC foams 3.2.1 PEDOT film electrodeposited on RVC 100ppi foam RVC foam is a sponge-like carbon material, which is solely composed of vitreous carbon and has quite a homogenous pore size distribution. RVC foam has a porous structure with a high void volume. This makes the electrodeposition of PEDOT onto RVC foam diverged from the case of a flat and smooth surface, like a GC electrode. The presence of the small voids affects the diffusion of the active species, like the monomer and enzyme, through the electrode material in the electropolymerization. This directly affects the growth of the polymer film, or in other words, the distribution of the film on the RVC foam. From the point of view of biocathode construction, an evenly distributed film over the entire porous structure is preferred, since the main motivation of utilizing the RVC foam is to make use of its large surface area per unit volume. To obtain a balance between the diffusion of monomer and enzyme molecules through the voids of the RVC foam material and the electropolymerization speed of PEDOT, an appropriate polymerization potential needs to be applied. Otherwise it is possible; for example, that the polymerization proceeds so fast that the PEDOT film preferably grows along the outermost surface of the foam to a thick film, instead of being polymerized into a well-distributed film within the entire pore structure. When the electropolymerization was performed under a comparatively low potential of 0.915 V, and a thickness of 2 mm was chosen for the RVC 100ppi electrode, the PEDOT film was able to grow evenly in the entire foam structure. When the RVC foam electrode was observed after the electropolymerization, a bluish-coloured PEDOT film was visible on the foam skeleton. In Fig. 2 , SEM images of the RVC foam electrode with PEDOT film polymerized on the foam surface (100 mC cm −3 ), are displayed. The average pore size of RVC 100ppi is about 230 μm. As seen in Fig. 2 b, a PEDOT film was deposited on the surface of the foam skeleton. When the polymer film was observed closely, its surface exhibited a rough and ‘grain-like’ morphology. 3.2.2 Fine-tuning the electrochemical performance of the biocathode RVC 100ppi/PEDOT-NO 3 / Th L, by optimizing the working parameters in the electropolymerization Th L was entrapped into the PEDOT conducting polymer film during the electropolymerization. Therefore, the amount of polymer generated and the concentration of enzyme in the electropolymerization electrolyte are the dominating parameters affecting the performance of a biocathode using entrapped Th L as the biocatalyst. Biocathodes were manufactured by electrochemically depositing 100 mC cm −3 PEDOT film on RVC 100ppi with varying concentration of Th L present in the electrolyte solution. The performance of the hereby obtained biocathodes, towards the reduction of oxygen, was examined by chronoamperometric measurements. The polarization curves for the different biocathodes can be seen in Fig. 3 a . The curve 1 in Fig. 3 a is the reference curve for PEDOT film generated on RVC 100ppi without any Th L entrapment. Without the presence of enzyme, there was no distinct reduction current observed except the contribution from the reduction of ABTS 2− . In curves 2, 3, and 4, variant concentrations of Th L were used in the electropolymerization. In all three cases, an apparent cathodic current was detected, resulting from the oxygen reduction catalyzed by Th L. The reduction of oxygen occurs at a potential of 0.66 V, which is quite close to the reversible O 2 /H 2 O half-cell potential at pH 4.5 (0.75 V vs Ag/AgCl(s)) and the cathodic current levels off at 0.45 V. The employment of Th L in the cathode renders it possible to achieve a high cell voltage for the O 2 -consuming biofuel cells, when used with a suitable anodic enzyme electrode construction. Furthermore, the plateau value of cathodic current density increased gradually with increasing enzyme concentration in the electrolyte. In Fig. 3 b, the Th L concentration used in the electropolymerization is plotted vs the steady current density generated at the potential of 0.45 V. A quasi-linear dependence was demonstrated, which indicates that the entrapped amount of Th L, linearly depended on the concentration of Th L in the electrolyte in the studied concentration range. When 19.5 μg ml −1 of Th L, the highest concentration studied in our experiments, was used in the electrolyte, the biocathode produced a steady current density, reaching above 1 mA cm −3 at 0.45 V. The current density is here reported vs the volume of RVC foam electrode. As shown in Fig. 1 b, when more polymer was generated, more Th L was entrapped into the PEDOT film. Additionally, the charge used for the electropolymerization was varied during the biocathode manufacturing, while the concentration of Th L (7.8 μg ml −1 ) in the electrolyte was kept constant. The amount of charge used in the electropolymerization is a parameter which directly reflects the PEDOT film thickness. The different polarization curves are displayed in Fig. 4 . It was found that when the used charge density was <110 mC cm −3 , the current density generated by the biocathode increased gradually when more polymer was generated onto RVC foam. However, the polarization curves in the cases of 167 mC cm −3 and 550 mC cm −3 are almost coincident with the polarization curve for 110 mC cm −3 . Hence, the current density did not change notably when the used charge density exceeded 110 mC cm −3 . Therefore, the thickness, corresponding to the deposition charge density of 110 mC cm −3 , can be considered as a critical point, within which oxygen can successfully diffuse into the film and interact with the active center of the immobilized Th L in the PEDOT film. Due to the oxygen diffusion problem, even though, the film can grow much thicker, only the immobilized Th L within the critical thickness is available for the substrate (O 2 ) and exhibits the catalytic activity on the oxygen reduction. 3.2.3 Working pH profile of the biocathode RVC 100ppi/PEDOT-NO 3 / Th L For an enzyme electrode, its working pH profile is a crucial issue, particularly, in a biofuel cell application, as one of its potential application areas is as a power source for the implantable devices. A neutral working pH is, thus, preferable due to the physiological working conditions. To determine the working pH profile for the biocathode and evaluate the effect of the immobilization on the working pH of Th L, the steady current density of the biocathode at 0.45 V was recorded using variant pH buffers as the electrolyte solutions. A plot of the steady current density vs the pH of the electrolyte solution is shown in Fig. 5 . It was found that the biocathode performance was sensitive to the variation in pH and the immobilized Th L had a very narrow working pH range with the optimal value at pH 4.2. The current density dramatically declined when pH increased: merely 60%, 40%, 23% or 6% of the current density was reserved when pH increased to 4.5, 5, 5.5, or 6, respectively. Compared with the diffusional Th L using electron-no-proton donor, ABTS, as the mediator [30] , the pH-optimum for the Th L immobilized in PEDOT film is shifted one unit more from pH 3.0–3.5 to pH 4.2. It is inferred that the micro-circumstance of Th L location is altered in its immobilization into the PEDOT film, which has an influence on the working pH profile of Th L. 3.2.4 Stability tests for the biocathode RVC 100ppi/PEDOT-NO 3 / Th L The chronoamperometric response of the freshly prepared biocathode at 0.45 V was recorded for 3 h in a quiescent 0.05 M pH 4.5 succinate buffer (10 ml) with 0.2 mM ABTS as mediator. The electrolyte was thoroughly purged with O 2 for half an hour before performing the measurement, to ensure the oxygen saturation at the beginning of the measurement. As can be seen in the chronoamperometric curve, shown in Fig. 6 , the current density declined gradually with prolongation of the measurement time. When comparing with the result measured in the fresh electrolyte solution after 1 day, it was found out that the declination of the current density was not merely caused by the leakage or denaturation of the Th L during the test. Instead, the results indicated that the declination of the current density was mainly induced by the depletion of oxygen from the electrolyte solution. To further evaluate the long-term stability of the immobilized Th L in the biocathode, the current density of the biocathode generated at 0.45 V is plotted vs the storage time in Fig. 7 . The results show that the catalytic activity of the immobilized Th L gradually declined during the storage under +4 °C in the succinate buffer. After 1 day, the biocathode retained 80% of its original activity. After 1 week, 50% was retained. Furthermore, only 30% of the original activity was preserved after 1 month of storage. The loss of enzyme activity is most likely caused by the leakage of laccase molecules from the accommodation sites in the PEDOT film. The pore size distribution could account for the rapid initial enzyme loss from the largest pores, followed by slow diffusion from smaller, deeper within the layer situated pores. 3.3 The use of Th L containing PANI ink in preparing biocathodes for biofuel cells The use of Th L in an ink-based conducting polymer immobilization matrix was additionally studied as this type of matrix would offer several benefits related to the manufacturing process and scalability of the biocathodes. PANI is an intensively studied conducting polymer, which is traditionally synthesized via chemical and electrochemical methods in a highly acidic medium by aniline oxidative polymerization. However, these traditional methods have several drawbacks such as the necessity of using strong acids and large amounts of chemical oxidants. These methods also require additional purification stages during the synthesis process. One alternative to these classical routes is an enzymatic polymerization of aniline, since this allows the process to be performed under mild conditions and can be considered as an environmentally friendly synthetic alternative. In this way it is also possible to obtain polymer, which is not contaminated with the decomposition products of the oxidizing agent and which has a high yield [31] . The use of laccase as biocatalyst for the aniline polymerization reaction allows avoiding problems related to the addition of supplementary components into the reaction mixture, since the only oxidizing agent, which is molecular oxygen, enters the reaction mixture from air. Further, laccases are relatively stable under low pH values and the only co-product of the reaction with laccases is water [32] . 3.3.1 Electrochemical evaluation and characterization of Th L containing PANI ink biocathodes Polarization curves of the biocathodes manufactured on carbon paper GDL35 AA (disc diameter 5 mm) by applying two different compositions of the Th L containing PANI ink (PANI ink 1 and 2) on the carbon paper template are shown in Fig. 8 . As can be seen in Fig. 8 , PANI ink 2, containing a higher concentration of Th L, displays a higher current density in the potential interval of 0.7–0.4 V vs Ag/AgCl(s). The current, however, decreases during the prolonged measurement in lower voltages, due to leaching of the PANI ink from the cellophane covered electrode assembly to the electrolyte solution. In Fig. 9 , different amounts of the Th L containing PANI ink 2, are applied on the carbon paper template and the response of the so obtained biocathodes is thereafter measured. It can be seen in Fig. 9 that the current density of the biocathode increases with the increasing amount of the Th L containing PANI ink 2. However, the leaching effect is similarly notable during this measurement as in the previous one. It can be noted that while this electrode assembly provides a similar measurement setup as was used with the PEDOT based biocathodes, it is clearly not the optimal for the PANI ink based biocathodes, where the leakage effect is more pronounced. Cyclic voltammograms for a biocathode manufactured on carbon paper GDL35 AA (disc diameter 5 mm) by applying 2.5 μl of the Th L containing PANI ink 2 are shown in Fig. 10 . The voltammograms are taken under argon and oxygen atmospheres and show the effect of oxygen on the electrochemical response of the biocathode. It is, thereby, shown that Th L immobilized into PANI ink also retains its catalytic activity towards the oxygen reduction into water and the current density of the so obtained Th L containing biocathode is controllable by adjusting the concentration of Th L in the PANI ink and the amount of PANI ink used. It can, thus, be concluded that Th L activated polymerization of PANI is applicable for the purpose of immobilizing biocatalyst in the construction of biofuel cell cathodes. 4 Conclusion In the present work, we have studied the utilization of porous carbon-based materials in combination with conducting polymers as matrices for enzyme immobilization in a biocathode design to improve the generated current density. In situ entrapment of enzyme molecules as dopants during the electropolymerization of EDOT was successfully used to immobilize Th L as the catalyst in the construction of an oxygen-consuming cathode for biofuel cell application. The immobilized Th L in PEDOT films still preserved its catalytic activity towards the oxygen reduction into water. RVC foam-based biocathodes exhibited a high cathodic open circuit potential and generated a steady-state current density with a magnitude of several hundred μA cm −3 , adjustable by varying the working parameters, like the electrodeposition charge density and Th L concentration used in the electrolyte. The thickness, corresponding to the deposition charge density of 110 mC cm −3 , is the critical thickness for successful oxygen diffusion into the polymer layer. The cathodic current density is rather sensitive to pH, and demonstrated its optima at pH 4.2. When pH increased to 4.5, 40% enzyme catalytic activity was lost. The biocathode retained 80%, 50%, and 30% catalytic activity after the storage in a +4 °C buffer solution for 1 day, 1 week and 1 month, respectively. In situ entrapment of Th L during the laccase activated polymerization of aniline using a chemical batch reaction method and simultaneously preparing a printable ink product was also successfully used to immobilize Th L as the catalyst in the construction of oxygen-consuming cathode for biofuel cell application. The immobilized Th L in PANI inks still preserved its catalytic activity towards the oxygen reduction into water. Further, the current density of the so obtained Th L containing biocathodes is controllable by adjusting the concentration of Th L in the PANI ink and the amount of PANI ink used. Nowadays, it is commonly recognized that the removal of a diffusional mediator is a crucial step for the miniaturization of the biofuel cells; otherwise the co-immobilization of the mediator is preferred in the biofuel cell structure designing. Our ongoing and future research is aiming to the direct electron transfer between the immobilized enzyme and the current collector, promoted by the integration of different nanosized constructive components into the 3-dimensional enzyme electrode designs. Acknowledgements Financial support from the 6th EU-framework program project ‘BIO-MEDNANO’ (STRP 017350) and the Finnish national project ‘Tekes-PEPSic’ (1681/31/07) is gratefully acknowledged and additionally Ms Xiaoju Wang would like to thank the Finnish Graduate School of Chemical Engineering for the financial support to her Ph.D. study. References [1] S.C. Barton J. Gallaway P. Atanassovv Chem. Rev. 104 2004 4867 4886 [2] R.A. Bullen T.C. Arnot J.B. Lakeman F.C. Walsh Biosens. Bioelectron. 21 2006 2015 2045 [3] J. Kim H. Jia P. Wang Biotechnol. Adv. 24 2006 296 308 [4] N. Mano F. Mao W. Shin T. Chen A. Heller Chem. Commun. 2003 518 519 [5] N. Mano F. Mao A. Heller J. Am. Chem. Soc. 125 2003 6588 6594 [6] N. Mano F. Mao A. Heller Chem. Biol. Chem. 5 2004 1703 1705 [7] I. Zawisza J. Rogalski M. Opallo J. Electroanal. Chem. 588 2006 244 252 [8] W. Nogala E. Rozniecka I. Zawisza J. Rogalski M. Opallo Electrochem. Commun. 8 2006 1850 1854 [9] J. Lim N. Cirigliano J. Wang B. Dunn Phys. Chem. Chem. Phys. 9 2007 1809 1814 [10] D. Ivnitski B. Branch P. Atanassow C. Apblett Electrochem. Commun. 8 2006 1204 1210 [11] Y. Yan W. Zheng L. Su L. Mao Adv. Mater. 18 2006 2639 2643 [12] F. Gao Y. Yan L. Su L. Wang L. Mao Electrochem. Commun. 9 2007 989 996 [13] K. Servat S. Tingry L. Brunel S. Querelle M. Cretin C. Innocent C. Jolivalt M. Rolland J. Appl. Electrochem. 37 2007 121 127 [14] P.N. Bartlett J.M. Cooper J. Electroanal. Chem. 363 1993 1 12 [15] W. Schuhmann Mikrochim. Acta 121 1995 1 29 [16] S. Cosnier Biosens. Bioelectron. 14 1999 443 456 [17] E. Simon C.M. Halliwell C.S. Toh A.E.G. Cass P.N. Bartlett Bioelectrochemistry 55 2002 13 15 [18] A. Ramanavicius K. Habermuller E. Csöregi V. Laurinavicius W. Schuhmann Anal. Chem. 71 1999 3581 3586 [19] L. Brunel J. Denele K. Servat K.B. Kokoh C. Jolivalt C. Innocent M. Certin M. Rolland S. Tingry Electrochem. Commun. 9 2007 331 336 [20] J.M. Friedrich C. Ponce-de-leon G.W. Reade F.C. Walsh J. Electroanal. Chem. 561 2004 203 217 [21] L. Groenendaal F. Jonas D. Freitag H. Pielartzik J.R. Reynolds Adv. Mater. 12 2000 481 [22] M. Lefebvre Z. Qi D. Rana P.G. Pickup Chem. Mater. 11 1999 262 268 [23] X. Wang P. Sjöberg-Eerola J.-E. Eriksson J. Bobacka M. Bergelin Synth. Met. 160 2010 1373 1381 [24] N. Duran M.A. Rosa L. Gianfreda Enzyme Microb. Technol. 31 2002 907 931 [25] G.T.R. Palmore H.H. Kim J. Electroanal. Chem. 464 1999 110 117 [26] S. Tsujimura H. Tatsumi T. Ikeda J. Electroanal. Chem. 494 2001 69 75 [27] S. Shleev A. Christenson V. Serezhenkov D. Burbaev A. Yaropolov L. Gorton T. Ruzgas Biochem. J. 385 2005 745 754 [28] A.V. Karamyshev S.V. Shleev O.V. Koroleva A.I. Yaropolov I.Y. Sakharov Enzyme Microb. Technol. 33 2003 556 564 [29] A.V. Streltsov O.V. Morozova N.A. Arkharova V.V. Klechkovskaya I.N. Staroverova G.P. Shumakovich A.Y. Yaropolov J. Appl. Polym. Sci. 114 2009 928 934 [30] M. Frasconi G. Favero H. Boer A. Koivula F. Mazzei Biochim. Biophys. Acta 1804 2010 899 908 [31] L.A. Samuelson Macromolecules 31 1998 4376 4378 [32] A.V. Strel’tsov O.V. Morozova N.A. Arkharova V.V. Klechkovskaya I.N. Staroverova G.P. Shumakovich A.I. Yaropolov Moscow Univ. Chem. Bull. 64 2009 107 110
更多查看译文
关键词
Enzymatic biofuel cell,Laccase,Biocathode,Polyaniline,Poly(3,4-ethylenedioxy-thiophene),Reticulated vitreous carbon foam
AI 理解论文
溯源树
样例
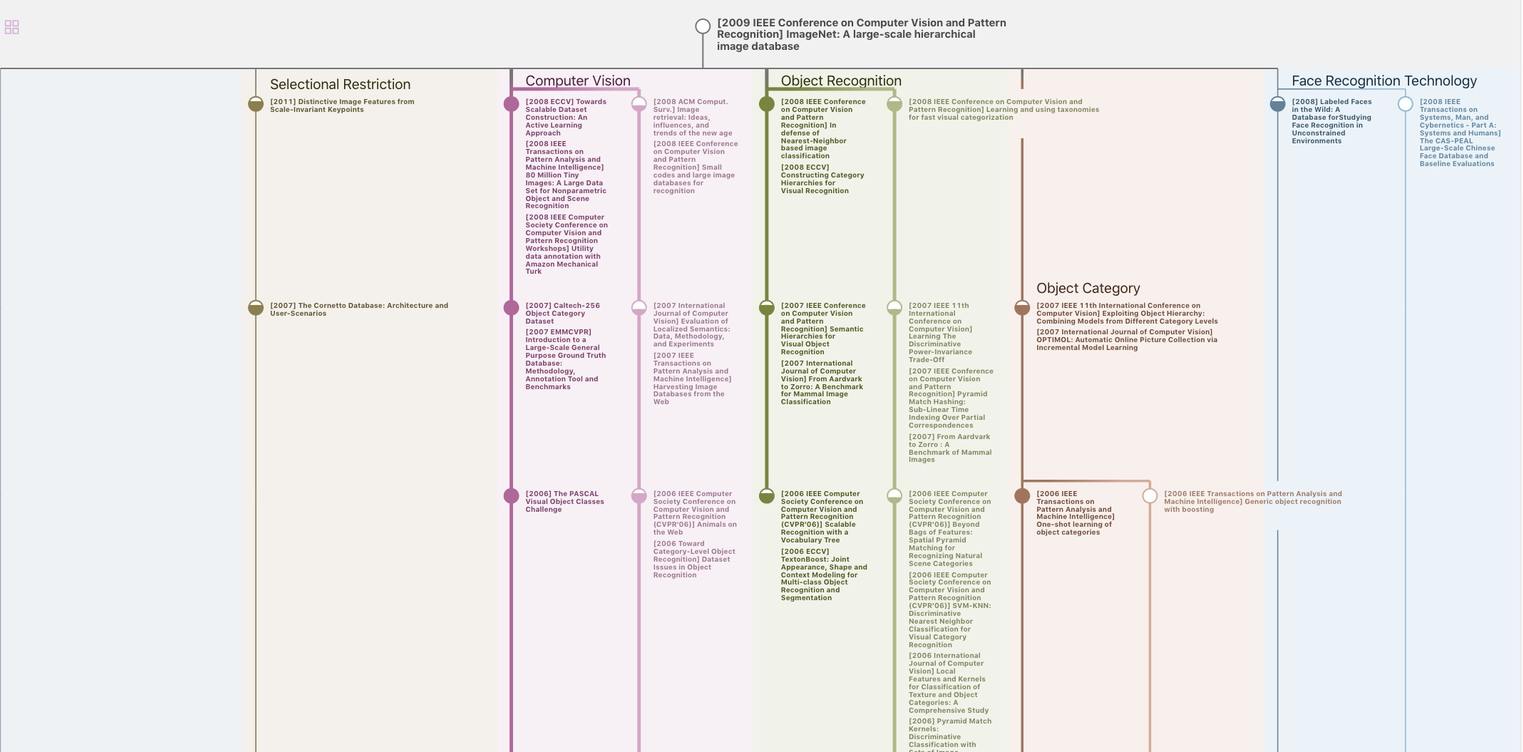
生成溯源树,研究论文发展脉络
Chat Paper
正在生成论文摘要