The effects of ozone and water exchange rates on water quality and rainbow trout Oncorhynchus mykiss performance in replicated water recirculating systems
Aquacultural Engineering(2011)
Abstract
Rainbow trout Oncorhynchus mykiss performance and water quality were evaluated and compared within six replicated 9.5 m 3 water recirculating aquaculture systems (WRAS) operated with and without ozone at various water exchange rates. Three separate studies were conducted: (1) low water exchange (0.26% of the total recycle flow) with and without ozone; (2) low water exchange with ozone versus high water exchange (2.6% of the total recycle flow) without ozone; and (3) near-zero water exchange (only backwash replacement) with and without ozone. Mean feed loading rates for WRAS operated at high, low, and near-zero exchange were 0.40, 3.98, and 55.9 kg feed/m 3 makeup water, respectively. Ozone significantly reduced total suspended solids, color, and biochemical oxygen demand and resulted in a significant increase in ultraviolet transmittance (%) ( P < 0.10). Ozone also created ambient water quality within low exchange WRAS that was comparable to that of WRAS operated at high water exchange ( P > 0.10). Additionally, dissolved copper and iron were significantly lower within WRAS operated with ozone ( P < 0.10). Dissolved zinc was also consistently lower in WRAS operated with ozone, but not significantly ( P > 0.10). In Studies 1 and 3, total ammonia nitrogen and nitrite nitrogen were slightly lower within the ozonated systems, but were not always significantly lower. In all studies, ozone did not prevent nitrate nitrogen accumulation. At the conclusion of Study 1, rainbow trout growth was significantly greater within low exchange WRAS operated with ozone ( P = 0.001). At the conclusion of Study 2, rainbow trout growth was similar between treatments ( P = 0.581), indicating that fish grew equally as well within ozonated WRAS operated at 1/10th the flushing rate as the non-ozonated and high flushing control systems. Overall, ozone created an improved water quality environment within low and near-zero exchange WRAS that generally resulted in enhanced rainbow trout growth rates, survival, feed conversion, and condition factor. Keywords Ozone Water reuse Recirculating aquaculture system Water quality Copper Heavy metals Waste metabolite accumulation Rainbow trout 1 Introduction A series of studies are being conducted to identify water quality parameters that could limit rainbow trout Oncorhynchus mykiss or Atlantic salmon Salmo salar performance (i.e. growth, health, welfare, and survival) within water reuse aquaculture systems (WRAS) that are operated at low water exchange with high feed loading rates ( Davidson et al., 2009; Good et al., 2009 ) or with high carbon dioxide concentrations ( Good et al., 2010 ). Davidson et al. (2009) pinpointed specific parameters that accumulated to potentially harmful levels when WRAS were operated at low exchange, i.e. 0.26% of the total recycle flow. Negative impacts on fish were not apparent; however, literature indicated that dissolved copper, fine and suspended solids, nitrate nitrogen concentrations, as well heterotrophic bacteria counts were a concern ( Davidson et al., 2009; Colt, 2006 ). Several other studies have examined accumulating water quality parameters within low exchange WRAS and their effect on the performance of various species. Martins et al. (2009a) concluded that ortho-phosphate-P, nitrate, and heavy metals (arsenic and copper) accumulated to levels that likely impaired the embryonic and larval development of common carp Cyprinus carpio . Deviller et al. (2005) attributed a 15% growth reduction in European sea bass Dicentrarchus labrax cultured within WRAS to an unknown “growth-inhibiting substance” and implied that metals accumulation could have been the cause. The accumulation of potentially harmful water quality concentrations in low exchange WRAS could represent a substantial barrier to the expanded utilization of this sustainable technology, particularly for species that require clean water such as salmonids. Therefore, methods that reduce and/or control accumulating water quality parameters within low exchange WRAS need to be evaluated. Previous research from the water treatment and aquaculture industries has shown that ozone can reduce and control a variety of water quality parameters, depending on where it is used and the dose of ozone applied. In varying applications, ozonation of water has been found to effectively reduce biochemical oxygen demand, chemical oxygen demand, dissolved organic carbon, color, nitrite, turbidity, total organic carbon, and/or total suspended solids ( Rosenthal and Kruner, 1985; Hozalski et al., 1995; Brazil, 1996; Summerfelt and Hochheimer, 1997; Summerfelt et al., 1997; Tango and Gagnon, 2003; Summerfelt et al., 2009a,b ). Ozone has also been used to control algae ( Rice et al., 1981; Plummer and Edzwald, 2002 ), improve micro-flocculation of fine particulates ( Rice et al., 1981; Rueter and Johnson, 1995 ), increase unit process efficiency ( Rosenthal and Otte, 1980; Paller and Lewis, 1988; Summerfelt et al., 1997 ), reduce heavy metals such as iron and manganese ( Rice et al., 1981; Langlais et al., 1991 ), and reduce bacterial populations depending on ozone dose, contact time, and operation with or without ultraviolet irradiation ( Summerfelt, 2003; Sharrer and Summerfelt, 2007; Summerfelt et al., 2008, 2009a,b ). Ozone has also been found to reduce off-flavor producing compounds such as MIB and geosmin in drinking water ( Terishima, 1988; Nerenberg et al., 2000; Park et al., 2007 ) using dosages that are orders of magnitude greater than those typically utilized in WRAS. However, the use of ozone at dosages that achieve water quality improvements within WRAS, but are insufficient to maintain an ozone residual or disinfect the water, has not proven effective at reducing off-flavor compounds ( Schrader et al., 2010 ). Ozone also reacts rapidly within water and produces few harmful byproducts in freshwater, where it forms dissolved oxygen as a reaction end product ( Summerfelt and Hochheimer, 1997; Summerfelt, 2003 ). A few studies have also indicated that the water quality improvements initiated by ozonation created a more optimal environment for growth and survival of various species cultured within WRAS. Suantika et al. (2001) found that ozonation ultimately improved rotifer production within a closed WRAS. In another study, ozonation of a seawater system culturing larval southern rock lobster Jasus edwardsii resulted in a significant decrease in bacterial populations and thus increased survival of lobster larvae ( Ritar et al., 2006 ). Brazil (1996) reported that hybrid striped bass Morone saxatilis x chrysops growth was improved in ozonated systems. The potential advantages of ozonation have also been demonstrated within recirculating systems used for salmonid culture. Bullock et al. (1997) reported that ozonation applied within a recirculating system reduced concentrations of suspended solids, dissolved organic carbon, color, and nitrite, and eliminated the need for chemotherapeutic treatment to control bacterial gill disease (BGD) in rainbow trout. The ozone dose applied during Bullock's study (0.025–0.039 kg ozone/kg feed) was not sufficient to kill Flavobacterium branchiophilum , the causative agent of BGD, providing <1 log reduction of the bacteria in the system water and on the gill tissue, but improved the culture environment to a point that rainbow trout were not impacted by the disease ( Bullock et al., 1997 ). Another study demonstrated the benefits of ozone for Atlantic salmon cultured within recirculating systems, including increased growth rates ( Sutterlin et al., 1984 ). The use of ozone in WRAS does not come without drawbacks. Excess ozone residual remaining in the culture water could cause significant harm or even catastrophic mortality to cultured species if not properly controlled ( Summerfelt et al., 2004a ). Ozone can also be hazardous to human health if air concentrations are not properly monitored and controlled ( Summerfelt et al., 2009a,b ). In-air monitors, alarms, and adequate ventilation systems are important to ensure worker safety when operating ozone. Lastly, ozone generation increases capital and operating costs. As long as the potential hazards related to ozone are controlled, the benefits of ozone relative to water quality and fish performance appear to outweigh the potential drawbacks. Ozone application within WRAS could be the key to optimal water quality control and fish performance within low and near-zero exchange WRAS operated with high feed loading. Thus, three studies evaluating the use of ozone within low and near-zero exchange WRAS are discussed in this paper. The primary objectives were: (1) to determine if ozone creates a more favorable water quality environment for salmonids as measured by rainbow trout growth and survival, and (2) to determine which water quality parameters are improved as a result of ozone addition. These results will provide important information regarding the feasibility of operating WRAS at low and near-zero water exchange, or as completely closed systems for the commercial production of rainbow trout and other salmonids. 2 Methods 2.1 Experimental treatments Rainbow trout performance and water quality criteria were evaluated and compared during three studies within six replicated water reuse aquaculture systems (WRAS), including WRAS operated with: (1) low water exchange rates with and without ozone; (2) low exchange rates with ozone versus high exchange without ozone; and (3) near-zero exchange with and without ozone. WRAS described as operating at “low” and “high” water exchange continuously replaced 0.26 and 2.6% of the total recirculating flow, respectively, while WRAS operated at “near-zero” water exchange replaced only the water that was lost as backwash or flushed from the radial flow settler. Makeup water was continuously added to the pump sump during Studies 1 and 2, but was introduced only as needed during Study 3 via a float valve located in the pump sump. Water replaced via the float valve during Studies 1 and 2 was not quantified because it represented <6% of the total daily makeup water addition within the “low” water exchange treatment and <1% of the total daily makeup water addition within the “high” water exchange treatment. Makeup flows were measured and calibrated several times per week during Studies 1 and 2. During Study 3, digital flow meters (Model C700, AMCO Water Metering Systems, Inc., Ocala, FL, USA) were installed on the makeup water lines of each WRAS to totalize the flow added via the float valve. During Study 3, periodic drum filter failures occurred within four of six WRAS, which resulted in increased and variable dilution amongst WRAS. Additionally, drum filter backwash spray was found to be added as additional makeup water to some WRAS and not others, also contributing to differences in dilution. Mean system hydraulic retention times relative to previously described flushing rates for the high, low, and near-zero exchange WRAS were approximately 0.67, 6.7 and 76 days, respectively. However, due to the variability in flushing during Study 3, mean hydraulic retention times for the individual WRAS varied from <10 days to as many as 196 days. 2.2 System description Six identical 9.5 m 3 WRAS ( Fig. 1 ), three per treatment, were used during each study and are described in detail in Davidson et al. (2009) . To summarize, each system recirculated 380 L/min (100 gpm) of water through a 5.3 m 3 dual drain culture tank, a radial flow settler, a microscreen drum filter with 60 μm screens, a fluidized sand biofilter, a geothermal heat exchanger, a carbon dioxide stripping column, and a low head oxygenator (LHO) ( Fig. 1 ). The recirculating flow exchanged the culture tank water volume once every 15 min. 2.3 Ozone Three WRAS were equipped with ozone generators (Model G22, Pacific Ozone Technology, Benicia, CA, USA). Approximately 1–6% of the >99% pure oxygen feed gas passing through the Corona discharge cell of each generator was converted to ozone and injected within the LHO. Ozone was monitored and controlled via oxidation reduction potential (ORP), measured in each culture tank just in front of the inlet flow structure with a differential ORP digital sensor equipped with a platinum electrode (Model DRD1R5, Hach Company, Loveland, CO, USA) and displayed by an SC100 Universal Controller (Hach Company, Loveland, CO, USA). During Studies 1 and 2, the SC100 was used to provide proportional-integral-derivative (PID) control of the ozone generator output in order to maintain an ORP set-point of 250 mV within the culture tank. During Study 3, the SC100 was used to provide on–off control of ozone generation to maintain an ORP set-point of 270–290 mV. ORP data was logged minute by minute over the duration of each study but was only available as raw data for Studies 2 and 3. The ORP set-points used during these studies were selected to prevent toxic ozone residuals from accumulating in the culture water in the absence of effective deozonation, such as the use of ultraviolet (UV) irradiation ( Summerfelt et al., 2004a ). The resulting ozone dose, which ranged from 20 to 25 g ozone/kg feed, was not intended to disinfect the water (i.e. reduce bacterial loads) at the ORP levels that were maintained, but was expected to improve general water quality. 2.4 Rainbow trout Study 1 —Rainbow trout (1000/tank), 74 ± 2 g, were stocked within each WRAS at a density of approximately 15 kg/m 3 and allowed eight weeks for acclimation and biofilter startup prior to ozone startup. The study began when ozone was turned on and was operating continuously 24 h per day. At the start of the study rainbow trout were 294 ± 3 g in systems operated with ozone and 296 ± 3 g in systems without ozone. Study 2 —Rainbow trout (1000/tank), 151 ± 3 g, were stocked at a density of approximately 30 kg/m 3 . Study 3—Rainbow trout (approximately 3600/tank), 18 ± 0 g, were stocked at a density of approximately 12 kg/m 3 . For Studies 2 and 3, a one-week acclimation period was used following stocking. Optimal nitrification had already been established across the biofilters when these studies began. 2.5 Photoperiod and feeding A constant 24-h photoperiod was provided for each study. Fish were fed equal rations with feeding events occurring every other hour, around the clock, using automated feeders (T-drum 2000CE, Arvotec, Huutokoski, Finland). Previous research ( Davidson et al., 2009 ) conducted within the same six WRAS indicated that the 24-h photoperiod and uniformly dispersed feeding events around the clock produced a relatively constant biological respiration rate as indicated by a nearly constant mean 24-h oxygen demand. Relatively constant daily water quality was required, because all water samples from the six systems could not be collected simultaneously. Feeding was estimated based on standardized feeding charts, as well as observations of feeding activity and wasted feed. If significant amounts of wasted feed and/or an obvious reduction in feeding response were observed, then feeding was adjusted for the respective WRAS. Thereafter, fish in each WRAS were fed to satiation but feeding was not necessarily equal between WRAS or between treatments. Feeding rates ranged from 1.0 to 3.0% of the fish biomass, depending on mean fish size. A standard slow-sinking trout diet (Zeigler Brothers, Inc., Gardners, PA, USA) with a protein: fat ratio of 42:16 was used throughout each study, with the exception of Study 3, during which a smaller pelleted 50:15 diet was fed during the first two weeks before switching to a 42:16 diet. 2.6 Water quality control and sampling protocols Alkalinity was maintained near 200 mg/L as CaCO 3 within low and near-zero exchange systems by adding sodium bicarbonate (NaHCO 3 ) in proportion to the daily feeding rate, i.e., 0.15 and 0.19 kg NaHCO 3 were added daily for every 1 kg of feed fed daily, respectively. Temperature was equalized amongst WRAS by adjusting water flow through the geothermal heat exchangers. The rapid culture tank hydraulic exchange rate (i.e., one exchange every 15 min) and the forced-ventilation stripping column were designed to maintain low carbon dioxide concentrations (typically <10 mg/L) that were equal amongst WRAS, even if feed loading levels differed slightly between culture tanks. The flow of oxygen feed gas to each LHO was manually adjusted when necessary to maintain dissolved oxygen concentrations in each culture tank at near 100% saturation for all WRAS. Water samples were manually collected from the side drain of each tank and tested for a variety of parameters ( Table 1 ). The majority of tests were carried out in-house by water chemistry staff. All water quality parameters were measured according to methods described in APHA (2005) and HACH (2003) . During a two-week period of “intensive sampling” when fish had reached near-maximum feed levels and densities (80 kg/m 3 ), water samples were collected on consecutive days to compare culture tank water quality between treatments; including samples for the analysis of 27 dissolved metals, which were conducted by the Cornell Nutrient Analysis Laboratory (Ithaca, NY, USA) ( Table 2 ). Water quality was also monitored on a weekly basis over the duration of each study. In addition, water samples were collected to evaluate bromide and the oxidized toxic compounds that can be formed during ozonation. During Studies 2 and 3 the following parameters were evaluated: bromide, bromine, bromate, and bromoform (within fish tissues). For the bromoform analysis, three fish were filleted from each WRAS and samples were homogenized per WRAS. Analyses were conducted by the following laboratories: bromine (Freshwater Institute), bromide and bromate (Test America, Nashville, TN, USA; and Broward Testing Laboratory, Ltd., Fort Lauderdale, FL, USA), and bromoform (Columbia Analytical Services, Inc., Kelso, WA, USA). The mean feed-specific NO 3 -N production constant, a nitrate (kg NO 3 -N/kg feed) was calculated for each of the WRAS by taking the difference in the NO 3 -N concentration entering and exiting each WRAS divided by its corresponding feed loading rate (and converting units). The mean feed-specific NO 3 -N production constant was calculated for each WRAS during each of the three studies when the WRAS were operated at near-maximum feeding rates. This calculation assumed that no denitrification or other NO 3 -N removal process other than dilution was involved. 2.7 Fish sampling protocols Fish were sampled for lengths and weights on a monthly basis. Sample size ranged from 50 to 120 fish and was calculated as follows: n = ( Z × (stdev. grams/accepted error grams)) 2 , where Z = 1.65 (relative to a 90% confidence interval) and accepted error was 5 g. Mortalities were removed and recorded daily to assess cumulative survival. During Study 3, mortalities related to a spike in ozone residual were excluded from the cumulative survival assessment, because the problem was associated with human error and not the specific treatment conditions. Fish were reared to a maximum density of 80 kg/m 3 and periodically thinned to approximately 50 kg/m 3 . Thermal growth coefficients (TGC), condition factor (CF), and feed conversion ratios (FCR) were calculated during each study and compared between treatments. Calculations are as follows: TGC = ((End Weight (1/3) − Start Weight (1/3) )/((Days Between × Mean Temp.) × 1000) CF = 100,000 × Weight/(Length) 3 FCR = Cumulative Feed Delivered to Tank/Fish Biomass Gain. where weight is in grams, length is in millimeters, and temperature is in °C. 2.8 Statistical analyses All parameters that were sampled during multiple events over time from the same location, such as water quality parameters measured during intensive sampling, as well as growth rates, were analyzed using multivariate repeated measures analysis of variance (MANOVA). Mean water quality data for the duration of each study, as well as metals data was compared between treatments using a Student's t -test. Normality was assumed for each test due to the relatively small sample size ( n = 3). For Study 3, water quality variables were analyzed for differences between treatments using analysis of covariance (ANCOVA), with feed loading per unit makeup water as the covariate, due to the unexpected differences in flushing rates measured amongst WRAS. However, the ANCOVA model violated assumptions for the analysis of most water quality parameters, with the exception of total ammonia nitrogen and nitrate nitrogen. Water quality parameters that did not meet the assumptions of ANCOVA were analyzed using a t -test during Study 3. Survival percentages were converted for statistical analysis using an arcsine square-root transformation as recommended by Sokal and Rohlf (1981) . A probability value ( α ) of 0.10 was used to determine significance for each statistical test as opposed to the traditional 0.05 due to sparsity of data, i.e. a relatively low n -value (three WRAS per treatment). Replication of WRAS was considered a unique advantage during these studies; however, it was not feasible to construct more than six WRAS to further increase statistical power. A higher α value is warranted under these circumstances, because the presence of just one outlier would increase variation and thus impact the significance of results, possibly resulting in Type II error. Statistical analyses were carried out using SYSTAT 11 software (Chicago, IL, USA) (2004). 3 Results and discussion 3.1 Metals/trace elements Concentrations of 11–14 dissolved metals/elements were detected during Studies 1–3 ( Table 3 ). The only metals/elements that were significantly different between treatments during Study 1 were copper and sulfur. Copper was significantly greater within WRAS without ozone ( P = 0.005), and sulfur was significantly greater in WRAS with ozone ( P = 0.051). During Study 2, significant differences were expected between high exchange WRAS without ozone and low exchange WRAS with ozone due to the 10-fold difference in flushing rate and thus greater dilution of the high exchange systems. The following metals/elements were significantly greater within the low exchange WRAS with ozone: copper, magnesium, phosphorous, potassium, sodium, strontium, and sulfur ( P < 0.10) ( Table 3 ). Barium and calcium were significantly greater within the high exchange WRAS ( P < 0.10). During Study 3, copper ( P = 0.005) and iron ( P = 0.044) were statistically greater within the near-zero exchange WRAS without ozone. Effects of ozone (copper) —The use of ozone caused a 3–4-fold reduction of dissolved copper within low and near-zero exchange WRAS during Studies 1 and 3. Dissolved copper measured within WRAS with and without ozone during Study 1 was 0.021 ± 0.008 and 0.064 ± 0.001 mg/L, respectively ( Table 3 ). During Study 3, dissolved copper within near-zero exchange WRAS operated with and without ozone was 0.041 ± 0.001 and 0.119 ± 0.008 mg/L, respectively ( Table 3 ), providing repeated evidence that ozone reduced dissolved copper. During Study 2, copper was greater within the low exchange WRAS with ozone (0.038 ± 0.004 mg/L) as compared to high exchange WRAS without ozone (0.014 ± 0.002 mg/L); however, the dissolved copper concentrations within the low exchange WRAS were most likely reduced by ozone, because these systems received a continuous makeup flow that was 10 times less than the high exchange WRAS. Thus, ozone likely controlled dissolved copper during Study 2 as well, but not as effectively as a 10-fold increase in system flushing. Effects of ozone (zinc) —Zinc was consistently lower within WRAS that used ozone, but not significantly. During Study 1 dissolved zinc was 0.005 ± 0.003 mg/L within low exchange WRAS without ozone and 0.001 ± 0.001 mg/L within low exchange WRAS with ozone ( Table 3 ). During Study 2 dissolved zinc was 0.011 ± 0.003 mg/L within the high exchange WRAS without ozone and 0.007 ± 0.002 mg/L within the low exchange WRAS with ozone. For Study 3 dissolved zinc was 0.128 ± 0.023 mg/L within the near-zero exchange WRAS without ozone as compared to 0.078 ± 0.003 mg/L within the near-zero exchange WRAS with ozone. Like copper, the accumulation of zinc within low or near-zero exchange WRAS tended to be reduced by ozonation. Effects of ozone (iron) —Dissolved iron was only detected in the culture water when WRAS were operated at near-zero exchange during Study 3. During Study 3, dissolved iron was 0.041 ± 0.013 mg/L within the near-zero exchange WRAS without ozone and 0.004 ± 0.002 mg/L within near-zero exchange WRAS operated with ozone. Therefore, ozone was also effective in reduction of dissolved iron, and therefore could be beneficial within aquaculture facilities that have relatively high levels of iron in their source water or within WRAS. Ozone's impact on metals and other elements has not been documented within aquaculture systems; however, research from the water treatment industry indicates that ozone can enhance removal of dissolved iron and manganese ( Rice et al., 1981; Langlais et al., 1991 ) and possibly other metals/elements due to its strong oxidizing capacity. The mechanism of removal of dissolved iron and manganese includes the oxidation and subsequent transformation of these metals from soluble to insoluble precipitates that can then be filtered ( Langlais et al., 1991 ). Little information is available regarding removal of copper and other metals/elements by ozonation; however, it is possible that a precipitating reaction occurred in the present studies between ozone and the metals that were removed (copper, iron, and zinc), similar to the reaction that was reported between ozone with iron and manganese. Precipitated metals could have chelated with organic molecules, become incorporated into biofilms, or become entrapped with the biosolids that were eventually removed from the WRAS via the tank bottom drain and radial flow settler or the drum filter along with the concentrated biosolids. Effects of ozone (potassium, magnesium, sulfur, phosphorus, and sodium) —Unlike copper, zinc, and iron, ozonation did not appear to decrease the accumulation of potassium, phosphorus, magnesium, sulfur, or sodium. Origin of metals/elements in WRAS —Dissolved metals were analyzed within the makeup water entering the WRAS from two sources: (1) a PVC pipe that flows to the pump sump as the primary makeup water and (2) a copper pipe that supplies high pressure backwash for the drum filter. Copper was typically undetectable within the makeup water entering through the PVC pipe (0.001 ± 0.001 mg/L); while dissolved copper was consistently detected within the water entering as backwash spray from the copper pipe (0.013 ± 0.002 mg/L). However, the majority of the water entering via the copper pipe was removed from the system with the backwashed solids and therefore was not the main source of the accumulating copper. Previous mass balance calculations ( Davidson et al., 2009 ) indicated that the majority of the accumulating dissolved copper was contributed by the feed. Other dissolved metals and trace elements were also likely introduced into the WRAS within the fish feed including: the majority of potassium, phosphorus, sulfur, and magnesium, which tended to accumulate in the water as system flushing was reduced. Sodium was introduced into the WRAS with daily addition of sodium bicarbonate (to replace alkalinity lost to nitrification) or occasional treatment of low-level bacterial gill disease with sodium chloride. Regardless of the origin of dissolved metals, it is apparent that metals, particularly copper, can accumulate to potentially harmful levels within low and near-zero exchange WRAS (without ozone) and should be monitored and controlled. 3.2 Nitrogenous waste Effects of ozone – Study 1 – There were no significant differences between low exchange WRAS operated with and without ozone for total ammonia nitrogen (TAN), nitrite nitrogen (NO 2 -N), or nitrate nitrogen (NO 3 -N) during the week of intensive sampling ( Table 4 ). TAN and NO 2 -N were slightly lower within the ozonated systems, but not significantly. Mean TAN levels between WRAS with and without ozone were 0.59 ± 0.03 and 0.53 ± 0.02 mg/L, respectively ( P = 0.109) ( Table 4 ). NO 2 -N levels in WRAS with and without ozone were 0.06 ± 0.01 and 0.05 ± 0.01 mg/L ( P = 0.782). Similar trends were observed for TAN and NO 2 -N over the study duration ( Table 5 ). Nitrate nitrogen was similar between WRAS with and without ozone, i.e. 90 ± 1 and 91 ± 1 mg/L, respectively, during the week of intensive sampling ( P = 0.623). However, NO 3 -N was significantly greater within the ozonated WRAS for the study duration ( P = 0.038), i.e., 84 ± 3 vs. 71 ± 1 mg/L in WRAS without ozone ( Table 5 ). The greater NO 3 -N concentrations within the ozonated WRAS for the study duration were attributed to slight differences in feeding between treatments. Slightly improved removal efficiency was observed across the biofilters in WRAS operated with ozone. Improved removal efficiency likely resulted due to cumulative water quality improvements initiated by ozone, especially the large reduction in carbonaceous biochemical oxygen demand (BOD) concentration entering the biofilter. Unit process removal efficiency measured during these studies will be described in another publication. Study 2 —Significant differences in water quality were expected because three WRAS were operated at high exchange and three at low exchange. Accordingly, TAN was significantly greater ( P = 0.073) within the low exchange WRAS with ozone (0.73 ± 0.04 mg/L) as compared to the high exchange WRAS with no ozone (0.55 ± 0.06 mg/L) during the week of intensive sampling, as well as for the study duration ( P = 0.005) ( Tables 4 and 5 ). Despite the difference in dilution between treatments, NO 2 -N was slightly greater, but not significantly, within the high exchange WRAS without ozone (0.17 ± 0.12 mg/L) as compared to the low exchange WRAS with ozone (0.13 ± 0.04 mg/L) during the week of intensive sampling ( P = 0.774) ( Table 4 ), as well as for the study duration ( P = 0.526) ( Table 5 ). The slightly lower NO 2 -N levels within the ozonated systems were attributed to ozone's ability to oxidize nitrite. The comparable TAN and NO 2 -N concentrations that were measured within the low exchange WRAS are indications of the effectiveness of the fluidized sand biological filter at converting TAN to NO 3 -N in an ozonated system, despite the 10-fold difference in system flushing rate between treatments. Nitrate nitrogen concentrations for WRAS operated at high exchange without ozone and WRAS operated at low exchange with ozone during the week of intensive sampling averaged 17 ± 1 and 108 ± 18 mg/L, respectively ( P = 0.008) ( Table 4 ). And, for the study duration, mean NO 3 -N levels were 13 ± 0 and 99 ± 7 mg/L, respectively ( P = 0.005). Because the makeup water supplied to these systems contained approximately 3 mg/L of NO 3 -N, there was a nearly 10-fold difference in mean concentrations of NO 3 -N that accumulated in the low and high flushing treatments over the duration of the study ( Table 5 ), i.e., [99 − 3]/[13 − 3] = 9.6:1, which is representative of the 10:1 difference in dilution between these treatments. Thus, over the entire study, NO 3 -N accumulation was directly proportional to the feeding rate and the mean hydraulic retention time of the WRAS, which did not provide a dedicated denitrification process. During the week of intensive sampling ( Table 4 ), the mean concentrations of NO 3 -N that accumulated in the low and high flushing treatments was only 7.5:1 (low:high); this discrepancy may have been created by passive denitrification, as discussed below (Study 3). Study 3 —TAN and NO 2 -N concentrations were slightly lower within ozonated WRAS, replicating trends observed during Study 1. TAN concentrations within the near-zero exchange WRAS with and without ozone during intensive sampling were 0.93 ± 0.01 and 1.23 ± 0.19 mg/L, respectively, but this difference was not statistically significant ( P = 0.528). TAN was also slightly lower, but not significantly, within the ozonated WRAS over the study duration, i.e. 0.72 ± 0.05 mg/L versus 0.92 ± 0.09 mg/L within the WRAS without ozone ( P = 0.123). NO 2 -N within near-zero exchange WRAS with and without ozone during intensive sampling was 0.08 ± 0.03 and 0.21 ± 0.05 mg/L, respectively ( P = 0.757). Over the study duration NO 2 -N was 0.12 ± 0.05 and 0.13 ± 0.01 mg/L, respectively ( P = 0.749). Failure of the WRAS 2 ozone generator, at one point, caused an increase in mean NO 2 -N for the ozonated WRAS. The increase in NO 2 -N was attributed to either an increase in the carbonaceous BOD and TSS concentrations entering the biofilter (after ozone generator failure) or the biofilter had become accustomed to NO 2 -N oxidation by ozone; therefore, when the ozone generator failed, sufficient nitrifying bacteria were not present to efficiently remove NO 2 -N. Nitrite nitrogen peaked to 0.88 mg/L in WRAS 2 during this period. Despite this anomaly, mean NO2-N was still slightly lower within the ozonated WRAS. Nitrate nitrogen accumulated to relatively high levels due to decreased water exchange during this study. Nitrate nitrogen within the near-zero exchange WRAS with and without ozone during intensive sampling was 373 ± 66 and 191 ± 28 mg/L, respectively ( Table 4 ) and was significantly different between treatments ( P = 0.048). Significant differences in NO 3 -N were not attributed to the treatment (i.e. ozone or no ozone), as ozone has not been shown to reduce NO 3 -N concentration in the literature. Instead significant differences were likely detected between treatments due to unexpected failures in drum filter flushing (explained in the Section 2 ) which created increased water exchange and dilution of NO 3 -N, primarily within WRAS that were operated without ozone. The drum filter failures and subsequent increased flushing occurred during weeks prior to intensive sampling. Over the study duration NO 3 -N within the near-zero exchange WRAS with and without ozone was 323 ± 87 and 171 ± 16 mg/L, respectively. Analysis of covariance, which was utilized to account for differences in flushing and feed loading rate between WRAS, indicated that there was not a significant difference between treatments for nitrate nitrogen over the study duration ( P = 0.644) ( Table 5 ). Using data collected during the period of near-maximum feed loading during all three studies, the mean feed-specific NO 3 -N production constant was found to decrease logarithmically as feed loading rate increased ( R 2 = 0.8591), i.e., a nitrate = −0.0041 × LN(Feed Loading Rate) + 0.0233, from a high of 0.026 kg NO 3 -N produced per kilogram feed fed at a feed loading of 0.61 kg/m 3 makeup flow to a low of 0.002 kg/kg at a feed loading rate greater than approximately 120 kg/m 3 makeup water ( Fig. 2 ). At the same time, the NO 3 -N concentration increased approximately logarithmically as feed loading rate increased ( Fig. 2 ; R 2 = 0.6318). Extrapolating this relationship to a single-pass system with a feed loading rate of approximately 0.01 kg/m 3 makeup water, a mean feed-specific NO 3 -N production constant of approximately 0.042 kg NO 3 -N would be produced per kilogram feed fed, which is close to the total ammonia nitrogen production rate expected per unit of feed consumed. Thus, some portion of the NO 3 -N that was produced was subsequently removed within the WRAS, and more NO 3 -N was removed as feed loading rate increased. Thus, passive denitrification or other NO 3 -N removal process occurred at higher feed loading rates (corresponding to longer system HRT) and with higher NO 3 -N concentrations. We suspect that passive denitrification occurred after NO 3 -N diffused deep enough into biofilms to effectively shelter the denitrifying bacteria from the aerobic conditions found elsewhere in the WRAS. 3.3 Alkalinity Mean alkalinity was significantly different between treatments over the duration of Studies 1 and 2 ( P = 0.004; 0.009), but was not different for Study 3 ( P = 0.169) ( Table 5 ). Alkalinity was generally lower within WRAS operated with ozone, but differences in alkalinity between treatments were attributed to slight variations in sodium bicarbonate addition. In low and near-zero exchange WRAS that use biofiltration, alkalinity is consumed during the nitrification process and must be supplemented to maintain homeostatic conditions ( Loyless and Malone, 1997 ). In fact, nitrification efficiency drops when alkalinity falls below 40–80 mg/L as CaCO 3 ( Paz, 1984; Biesterfeld et al., 2003 ). Chen et al. (2006) recommends maintaining an alkalinity of 200 mg/L CaCO 3 in WRAS operated with minimal water exchange for optimal biofilter performance. During Studies 1 and 2, approximately 0.15 kg of sodium bicarbonate/kg feed was added within WRAS operated at low exchange to maintain alkalinity near 200 mg/L. During Study 3, approximately 0.19 kg of sodium bicarbonate/kg feed was added within near-zero exchange WRAS to maintain alkalinity near 200 mg/L. 3.4 Total suspended solids Effects of ozone – Study 1 – Total suspended solids (TSS) within WRAS operated with ozone were significantly lower during the week of intensive sampling (4.7 ± 0.6 mg/L) and for the study duration (3.4 ± 0.4 mg/L) as compared to WRAS operated without ozone during intensive sampling (9.7 ± 1.4 mg/L) and for the study duration (8.7 ± 1.8 mg/L) ( P = 0.032; 0.001) ( Tables 4 and 5 ). Thus, ozone effectively reduced TSS within low exchange WRAS. Study 2 —TSS was significantly greater within WRAS operated at low exchange with ozone (5.1 ± 0.3 mg/L) vs. WRAS operated at high exchange without ozone (2.8 ± 0.2 mg/L) during the week of intensive sampling ( P = 0.003) ( Table 4 ). Over the study duration, TSS within the low exchange WRAS with ozone averaged 4.6 ± 0.5 mg/L, while TSS within WRAS operated at high exchange without ozone averaged 3.4 ± 0.1 mg/L ( P = 0.107) ( Table 5 ). Differences in TSS during Study 2 must be kept in perspective because of the 10-fold difference in water flushing rate between treatments. Ultimately, ozone produced mean TSS concentrations within low exchange WRAS that were quite low and similar to WRAS operated with 10 times more water exchange. Study 3 —Mean TSS concentrations during the week of intensive sampling for near-zero exchange WRAS with and without ozone were 3.5 ± 0.5 and 18.2 ± 5.9 mg/L, respectively ( P = 0.069) ( Table 4 ). TSS levels over the study duration for WRAS operated with and without ozone were 3.5 ± 0.6 and 18.9 ± 1.1 mg/L, respectively ( P = 0.001) ( Table 5 ). Thus, TSS concentrations were significantly lower within ozonated WRAS during intensive sampling and over the study duration, indicating that ozone significantly reduced TSS concentrations within near-zero exchange WRAS. Maximum TSS concentrations measured within the near-zero exchange WRAS with and without ozone were 14.6 and 63.3 mg/L, respectively. The significantly lower TSS concentrations within the ozonated WRAS are even more impressive when viewed in light of the unexpected differences in flushing rate. By chance, two of three systems operated with ozone (WRAS 2 and 6) were the least diluted systems and thus had much greater feed loading rates than other WRAS ( Table 6 ). As a result, mean feed loading rates for WRAS operated with and without ozone were 74 kg feed/m 3 makeup water/day vs. 38 kg feed/m 3 makeup water/day, respectively over the study duration; and 98 kg feed/m 3 makeup water/day vs. 76 kg feed/m 3 makeup water/day, respectively, during intensive sampling. Thus, ozonation dramatically reduced TSS concentrations in comparison to WRAS operated without ozone, despite working against much greater feed loading rates. TSS removal via ozonation has been previously demonstrated within WRAS. Summerfelt et al. (1997) observed a 35% reduction of TSS within a WRAS culturing rainbow trout in cross-flow raceways. In a much larger circular-tank-based WRAS, Summerfelt et al. (2009a,b) found that mean TSS concentrations within the culture tank could be reduced approximately 38–48%, from 4.0 mg/L (when the system water was not ozonated) to 2.1–2.5 mg/L, when ozone was applied. Tango and Gagnon (2003) found that ozone removed 40–50% of TSS within a marine recirculating system culturing Atlantic halibut Hippoglossus hippoglossus. Ozone causes micro-flocculation of fine solids ( Maier, 1984 ), which leads to increased removal efficiency across solids removal devices. TSS removal efficiency during the present studies indicated that solids removal across the radial flow settler and drum filter was greater within WRAS operated with ozone, indicating that ozone likely caused flocculation of smaller particles and subsequent removal within these unit processes. Effects of ozone — Fine particles —Analysis of particle counts and particle size distributions supports the theory of solids micro-flocculation and removal. Particle size distribution of the culture water during Study 1 ( Fig. 3 ) was consistent with previous research, which reported that the majority of suspended solids present in WRAS are ≤20 μm ( Patterson et al., 1999; Patterson and Watts, 2003; Chen et al., 1993 ). Mean total particle counts (2–60 μm) of samples collected for the duration of Study 1 were four times greater within WRAS without ozone (19,749 counts/mL) as compared to WRAS with ozone (4786 counts/mL). WRAS operated with ozone had substantially less fine particles for all size ranges ( Fig. 3 ). 3.5 Carbonaceous biochemical oxygen demand Effects of ozone – Study 1 – Carbonaceous BOD within low exchange WRAS with and without ozone averaged 1.8 ± 0.2 and 4.7 ± 0.9 mg/L, respectively, during the week of intensive sampling and carbonaceous BOD was significantly lower within WRAS operated with ozone ( P = 0.034). Carbonaceous BOD was also significantly lower within ozonated WRAS over the study duration ( P = 0.062) ( Table 5 ). Total organic carbon (TOC) and dissolved organic carbon (DOC) concentrations measured during Study 1 correlated with carbonaceous BOD results, as lower TOC and DOC concentrations were measured within WRAS operated with ozone ( Tables 4 and 5 ). Study 2 —Carbonaceous BOD was similar between treatments during the week of intensive sampling and over the study duration ( P = 0.431; 0.116) ( Tables 4 and 5 ). During the week of intensive sampling, carbonaceous BOD within the high exchange WRAS without ozone was 4.1 ± 0.7 mg/L, and carbonaceous BOD within the low exchange WRAS with ozone was 4.7 ± 0.3 mg/L. Although significant differences between treatments were not detected, these results are quite noteworthy because carbonaceous BOD concentrations within the low exchange WRAS with ozone were similar despite operation with 10 times less water exchange than the high exchange WRAS. Study 3 —Results indicated that ozone effectively reduced the accumulation of carbonaceous BOD in the WRAS. Mean carbonaceous BOD concentrations were significantly greater within WRAS operated without ozone during the week of intensive sampling and over the study duration ( P = 0.079; 0.096) ( Tables 4 and 5 ). When WRAS were operated near-maximum feed loading, carbonaceous BOD concentrations in near-zero exchange WRAS operated with and without ozone averaged 4.3 ± 0.5 and 18.9 ± 6.2 mg/L, respectively. The results from these studies indicated that ozone can substantially reduce carbonaceous BOD concentrations within WRAS, thus aiding in the optimization of the nitrification process. Carbonaceous BOD and TSS reduction via ozonation were likely related because a large portion of organic matter existed as measurable TSS. 3.6 Total heterotrophic bacteria plate count The total heterotrophic bacteria plate counts that were quantified during these studies represent bacteria present within the sampled WRAS water that were able to colonize on the agar plates used for analysis. Thus, the total heterotrophic bacteria plate count data does not encompass all of the waterborne bacteria within the culture systems, but does provide a generalized comparison of total heterotrophic plate counts between treatments. Effects of ozone – Study 1 – WRAS operated with low water exchange without ozone had approximately 2000-fold (3 log 10 ) more heterotrophic bacteria counts as compared to low exchange WRAS operated with ozone, i.e. 2.0 × 10 5 vs. 92 counts/mL ( Table 5 ). Despite this large disparity, a statistical difference was not detected due to widely variable heterotrophic counts between WRAS ( P = 0.240). The mean bacteria count for the WRAS without ozone was elevated (5.6 × 10 5 counts/mL) due to a four-week period during which bacteria counts exploded in this treatment. This increase in bacteria did not occur within the low exchange WRAS operated with ozone (105 counts/mL). The authors hypothesize that the increase in bacteria within the low exchange WRAS without ozone could have resulted due to a turnover of bacteria populations within the biofilters. Aside from this four-week period, mean heterotrophic bacteria counts within the low exchange WRAS without ozone were much lower, i.e. 641 counts/mL, but were still approximately seven times greater than the bacteria levels observed in the WRAS with ozone. The ozone dose applied during these studies was not strong enough for disinfection, but other water quality improvements initiated by ozone, such as reduction of TSS and carbonaceous BOD, created an environment that was not as conducive to bacterial proliferation. Study 2 —Heterotrophic bacteria counts for the high exchange WRAS operated without ozone and the low exchange WRAS operated with ozone were 117 ± 23 and 114 ± 19 counts/mL ( Table 5 ), respectively, and were not significantly different ( P = 0.933). Therefore, ozone created ambient water quality at low exchange that was similar to a high exchange WRAS, with relatively low heterotrophic bacteria counts. Study 3 —Heterotrophic bacteria counts within the culture water of near-zero exchange WRAS operated with and without ozone were 77 ± 17 and 825 ± 407 counts/mL ( Table 5 ). Despite the large disparity between treatments, a statistical difference was not detected due to variability in bacteria counts amongst WRAS operated without ozone ( P = 0.205). However, the results were consistent with the other studies indicating that ozone indirectly minimized heterotrophic bacteria. 3.7 Visual observations, UV transmittance, and color The reduction of TSS, carbonaceous BOD, bacteria, TOC, DOC, and refractory organic molecules via ozonation visually resulted in a culture environment with very clear water. In WRAS operated at low and near-zero exchange with ozone, the fish could easily be observed from above or through an observation window, while in WRAS operated at low and near-zero exchange without ozone, fish could barely be seen ( Fig. 4 ). These differences were reflected in measurements of ultraviolet (UV) transmittance and true color over the duration of each study. Effects of ozone — UV transmittance —UV transmittance is a measurement of the penetration of ultraviolet irradiation through a water sample. Mean UV transmittance for WRAS operated at low exchange with and without ozone was 82 ± 0 and 60 ± 1%, respectively, for the duration of Study 1 ( P = 0.000) ( Table 5 ). During Study 2, UV transmittance was significantly lower within WRAS operated at low exchange with ozone (77 ± 1%) as compared to WRAS operated at high exchange without ozone (89 ± 0%) ( Table 5 ), but this was partly due to the 10-fold difference in dilution between treatments ( P = 0.017). UV transmittance during Study 3 measured within near-zero exchange WRAS operated with and without ozone was 66 ± 4 and 30 ± 2% ( Table 5 ), respectively ( P = 0.002). In summary, ozone significantly increased UV transmittance when systems were operated at comparable flushing rates. Effects of ozone — Color —True color was significantly lower within the ozonated WRAS for Studies 1, 2, and 3 ( P = 0.000; 0.022; 0.026). During Study 1, color measured in low exchange WRAS with and without ozone averaged 4 ± 0 and 53 ± 2 Pt-Co units, respectively ( Table 5 ). During Study 2, color measured within the high exchange WRAS without ozone averaged 12 ± 1 Pt-Co units, while color within low exchange WRAS with ozone averaged 5 ± 1 Pt-Co units ( Table 5 ). Ozone also dramatically reduced color during Study 3. Mean color measured within near-zero exchange WRAS with ozone was 5 ± 1 Pt-Co units, while mean color within near-zero exchange WRAS without ozone was 157 ± 25 Pt-Co units. UV transmittance and true color measurements from all three studies demonstrated ozone's ability to provide clear water, which could be advantageous for both the fish and the fish farmer. A culture tank with clear water enhances the ability of the fish to see, feed optimally, and grow ( Sigler et al., 1984 ) and also allows the farmer to better observe fish health, behavior, and feeding activity ( Christensen et al., 2000 ). Increased UV transmittance in ozonated water allows for increased bacterial inactivation by UV and use of lower UV dosages to achieve the same level of disinfection. 3.8 Ozone residual, ORP, and ozone byproducts Oxidation reduction potential (ORP) was measured during each study as an indirect measure of ozone residual. Bullock et al. (1997) suggested that an ORP set-point of 300 mV was safe for rainbow trout, and Summerfelt et al. (2009a,b) reported that dissolved ozone concentrations were 0 ppb at an ORP up to 340 mV. ORP levels were maintained very close to the target levels (250 mV for Studies 1 and 2; 270–290 mV for Study 3) under most circumstances ( Fig. 5 ). However, during Studies 1 and 2, multiple events occurred during which the SC100 Universal Controller's PID control loop severely overshot the set-point when auto-tuning after restart, allowing ORP to spike to 100–300 mV beyond the target set-point ( Fig. 5 , Study 2). Several ORP spikes (≥400 mV) indicated potentially dangerous levels of ozone residual in the culture water, including a few during Study 2 that resulted in low-level mortality. During Study 3, ORP was controlled via on/off set-points programmed within the SC100 Universal Controller to maintain an ORP of 270–290 mV. One major spike occurred during Study 3 within WRAS 3 (ORP = 850 mV; Fig. 5 ) that resulted in significant fish mortality, but this spike was attributed to human error, i.e., the control function was manually turned off and not turned back on until too late. Not withstanding human error, on/off ORP control via the SC100 unit proved to be a more stable ozone control method that was safer for fish. Bromide ions (Br − ) are naturally occurring in seawater and many freshwater sources or can be present as impurities within salts such as sodium chloride ( Grguric et al., 1994 ). Ozone reacts very little with chloride, but its reaction with bromide can form relatively toxic residuals, including bromine and bromate (BrO 3 –) ( Steslow, 1991; Grguric et al., 1994; Tango and Gagnon, 2003; Tanaka and Matsumura, 2002, 2003 ). Bromide measured during Study 2 by Test America (Nashville, TN) using a MDL of 1 mg/L was undetectable for all six WRAS. However, results from the same sampling event that were sent to Broward Testing Laboratory (Ft. Lauderdale, FL, USA), which used an analytical technique with an MDL of 0.005 mg/L, indicated mean bromide levels of 0.020 ± 0.003 mg/L in the high exchange WRAS without ozone and 0.022 ± 0.007 mg/L (excluding WRAS 6) in the low exchange WRAS operated with ozone. Bromide within WRAS 6 was undetectable during this single sampling event, possibly indicating that bromide had been oxidized to bromine or bromate in this system. Natural salts containing bromide could have been present at low concentrations within the makeup water or bromide could have entered the WRAS as a contaminant within the sodium bicarbonate that was added daily to control alkalinity, or the sodium chloride that was added as a chemotherapeutic treatment as needed to counter low-level bacterial gill disease (BGD). Sodium chloride was added to the non-ozonated WRAS much more frequently than to the ozonated WRAS, due to higher incidence of BGD. Bromate was non-detectable in all WRAS samples at a MDL of 0.001 mg/L, with the exception of the single sample from WRAS 6 (low exchange with ozone), which was 0.056 mg/L. This sample was taken shortly after the PID control loop for ORP had malfunctioned in WRAS 6, causing ORP in the culture tank to increase to at least 388 mV ( Fig. 5 ). However, this measured bromate concentration was approximately 1000-times less than the concentration that Hutchinson et al. (1997) determined to be acutely toxic to rainbow trout. Bromine concentrations measured during Study 2 were less than or equal to the MDL's for the conducted assays: i.e. ≤0.03 mg/L. Supporting this MDL was the 0.02 ± 0.01 mg/L bromine concentration measured in the three WRAS where no ozone was added and bromine could not be present. During Study 3, the mean concentrations of bromide and bromate within the ozonated WRAS were 0.050 ± 0.010 mg/L and 0.023 ± 0.040 mg/L, respectively. Thus, detectable bromate concentrations were measured within each of the three WRAS that were operated with ozone. Unfortunately, in hind sight, samples from the non-ozonated WRAS were not sent for analysis to test the analytical method against the non-ozonated control condition. As previously mentioned, these levels of bromate are far below those levels reported to be acutely toxic. Bromoform was not detected (<0.050 mg/L MDL) within any fish tissue samples during Study 2 or 3. 3.9 Controlled water quality parameters Dissolved oxygen, carbon dioxide, and temperature were controlled throughout Studies 1–3 and therefore were similar between treatments for each study ( Tables 4 and 5 ). A significant difference for oxygen was detected between treatments during Study 2 for the study duration ( P = 0.006) ( Table 5 ), but the difference was only 0.2 mg/L, which was inconsequential to fish health or performance. 3.10 Growth, feed conversion, condition factor, and survival 3.10.1 Study 1 Rainbow trout growth was significantly greater within low exchange WRAS operated with ozone at the conclusion of Study 1 ( P = 0.001). Mean final weights for low exchange WRAS operated with and without ozone were 1161 ± 6 and 993 ± 12 g, respectively, four months after initiation of treatments ( Fig. 6 ). Mean thermal growth coefficients calculated for the study duration also reflected significantly faster growth rates for WRAS operated with ozone, i.e. 2.13 ± 0.01 vs. 1.83 ± 0.03 within WRAS without ozone ( P = 0.006). Additionally, repeated measures analysis indicated a highly significant difference relative to a time × treatment interaction ( P = 0.000) indicating that differences in mean fish weight occurred through time between the two treatments. Subsequent t -tests revealed that rainbow trout within the ozonated WRAS were significantly larger than trout in WRAS without ozone only one month after treatments were initiated. Fig. 6 illustrates the divergence of growth curves following the pre-study acclimation and set-up periods during which rainbow trout weights were equal. Feed conversion ratios indicated that trout in WRAS with ozone generally consumed feed more efficiently than trout cultured in WRAS without ozone ( Fig. 7 ). One month after initiation of treatments, substantial amounts of uneaten feed were observed within the radial flow settlers for WRAS operated without ozone. The increase in uneaten feed in WRAS without ozone coincided with the slower growth that occurred over the first month of the study. Feed conversion ratios for WRAS with and without ozone over the first month of ozone operation were 1.15 ± 0.06 and 1.38 ± 0.09, respectively ( P = 0.090) reflecting the increase in uneaten feed in WRAS without ozone ( Fig. 7 ). After the first month of the study, feeding was reduced to minimize uneaten feed, thus FCR's did not vary as much between treatments thereafter. However, overall FCR's were still slightly better (but not significantly) for fish cultured in WRAS with ozone. Cumulative FCR's for fish cultured in WRAS with and without ozone were 1.41 ± 0.03 and 1.51 ± 0.04, respectively ( P = 0.146) ( Fig. 7 ). Condition factor at the conclusion of the study was significantly greater for WRAS operated with ozone, 2.03 ± 0.01, versus WRAS without ozone, 1.95 ± 0.02 ( P = 0.011) ( Fig. 8 ). The greater condition factors for the rainbow trout from the ozonated WRAS appear to be due to slightly greater weight for a given length. Rainbow trout length for WRAS with and without ozone was 384 ± 1 and 370 ± 1 mm, respectively ( P = 0.001). A greater condition factor can be beneficial to a producer, because it typically indicates increased girth and potentially increased fillet yield. Survival calculated over the study duration for WRAS with and without ozone was 99.3 ± 0.2 and 98.3 ± 0.5%, respectively ( P = 0.113). Although a significant difference was not detected between treatments relative to survival, it is worth noting that ozonated WRAS had approximately half the mean cumulative mortalities as compared to WRAS without ozone, i.e. 7 ± 2 versus 17 ± 5 mortalities, respectively. 3.10.2 Study 2 Rainbow trout growth was similar between treatments throughout the duration of Study 2. At the conclusion, mean rainbow trout weights in WRAS operated at high exchange without ozone were 1379 ± 38 g versus 1348 ± 72 g in WRAS operated at low exchange with ozone ( Fig. 6 ). Repeated measures analysis indicated that there was not a difference between treatments ( P = 0.581) or a time × treatment interaction ( P = 0.991), indicating that growth rates were similar throughout the study. Mean thermal growth coefficients for the high exchange WRAS without ozone and the low exchange WRAS with ozone were 2.58 ± 0.05 and 2.54 ± 0.10, respectively, reflecting equal growth rates ( P = 0.790). Thus, rainbow trout performance within the low exchange WRAS with ozone was similar to that of a high exchange system without ozone, despite the 10-fold difference in flushing rate. Feed conversion ratios were also similar between treatments ( Fig. 7 ). The cumulative FCR for fish cultured in the low exchange WRAS with ozone averaged 1.52 ± 0.09 as compared to 1.43 ± 0.05 for fish cultured in the high exchange WRAS without ozone ( P = 0.254) ( Fig. 7 ). Condition factor at the conclusion of Study 2 was significantly greater for WRAS operated at low exchange with ozone, 2.10 ± 0.04, versus WRAS operated at high exchange without ozone, 1.87 ± 0.01 ( P = 0.021). Fig. 8 shows that the divergence in condition factor began very early in the study. The difference in condition factor was not related to differences in fish weight, because weights were equal, but instead was attributed to a difference in fish length. Mean fish length at the conclusion of the study for WRAS operated at high exchange without ozone was 418 ± 3 mm, but only 399 ± 5 mm within low exchange WRAS with ozone ( P = 0.044). We hypothesize that the difference in fish length and thus condition factor was related to a difference in fish swimming speed/exercise that was observed between treatments. Rainbow trout within the low exchange WRAS with ozone were consistently observed swimming forward at a much greater speed (i.e. 1.40 ± 0.06 body lengths/s) compared to fish cultured within WRAS operated at high exchange without ozone which generally held position in the water column, resulting in a swimming speed of 0.65 ± 0.18 body lengths/s ( P = 0.041). Note that swimming speeds were measured by timing fish as they swam past marked tank locations and then by factoring in the rotational velocity of water circling the tank, as described in Davidson et al. (under review) . A potential relationship was observed between rainbow trout swimming speed and certain constituents within water, such as nitrate nitrogen and dissolved potassium. Specifically, something in the water that was not related to ozone was causing sublethal effects that included abnormal swimming behavior and an increased incidence of spinal deformities. These finding are reported elsewhere ( Davidson et al., under review ). Rainbow trout survival was similar between the two treatments, during Study 2. Survival within WRAS operated at low exchange with ozone was 93.3 ± 1.6% and 93.1 ± 0.5% in WRAS operated at high exchange without ozone ( P = 0.787). 3.10.3 Study 3 Repeated measures analysis indicated that rainbow trout growth was similar between WRAS with and without ozone ( P = 0.267) and a sample x treatment interaction indicated that differences in rainbow trout weights did not occur between treatment during any sampling point during the study ( P = 0.196) At the conclusion of Study 3, rainbow trout within the near-zero exchange WRAS with and without ozone were 206 ± 14 and 180 ± 10 g, respectively ( Fig. 6 ). Mean thermal growth coefficients for fish cultured within near-zero exchange WRAS with and without ozone were 1.79 ± 0.07 and 1.66 ± 0.07, respectively ( P = 0.257). Feed conversion ratios were slightly better for WRAS operated at near-zero exchange with ozone, but not significantly ( P = 0.339). Mean FCR's over the study duration for the near-zero exchange WRAS with and without ozone were 1.30 ± 0.07 and 1.42 ± 0.08, respectively ( Fig. 7 ). Rainbow trout condition factor at the conclusion of the study was significantly greater within the near-zero exchange WRAS with ozone, i.e. 1.74 ± 0.07, as compared to the near-zero exchange WRAS without ozone, 1.40 ± 0.02 ( P = 0.034) ( Fig. 8 ). Although a statistical difference does exist between treatments, the authors do not believe that this difference is directly related to treatment. Instead, condition factor appears to correlate with differences in swimming behavior and the accumulation of certain constituents within the recirculated water that existed between treatments, as was also observed during Study 2 ( Davidson et al., under review ). Rainbow trout survival was similar between the WRAS operated at near-zero exchange with and without ozone. Survival within WRAS operated at near-zero exchange with and without ozone was 88.8 ± 3.3% and 94.5 ± 0.5%, respectively ( P = 0.229). It is important to note that the mortality event that occurred due to an ozone spike created by human error (described in Section 3.9 ) was excluded from the cumulative survival assessment. Although survival was lower for the ozonated WRAS, this difference was most likely not related to the ozone treatment, but instead to unexpected differences in flushing rates and the corresponding differences in water quality. For example, survival within one WRAS operated with ozone that had a HRT <10 days was 95.1%, while survival within WRAS operated with ozone with HRT's ≥94 days had cumulative survival of 83.8 and 87.6%. Thus, the overall decreased survival for WRAS operated with ozone was more likely related to some aspect of the water quality, because two of three ozonated WRAS had retention times ≥94 days and thus substantially greater concentrations of other parameters. 4 Summary and conclusions These studies indicated that the use of ozone within low and near-zero exchange water recirculating systems significantly improved a variety of water quality conditions. Ozone effectively reduced TSS, carbonaceous BOD, and color, and resulted in a significant increase in ultraviolet transmittance. As a result of the improved water quality conditions, total heterotrophic bacteria counts were also lower within ozonated WRAS. Most notably ozone effectively reduced accumulating metals within low and near-zero exchange WRAS, particularly copper. Ozone also reduced other potentially toxic metals, including zinc and iron. During Study 2, ozone created water quality within low exchange WRAS that was similar to high exchange WRAS that were operated with 10 times greater water exchange; and, during Study 3, ozone created lower concentrations of most water quality parameters including copper, iron, TSS, and BOD, even within WRAS that had retention times ≥94 days as compared to WRAS operated without ozone with retention times of approximately 40 days. Overall, ozone created a more optimal water quality environment that generally led to increased growth, survival, feed conversion, and condition factor of rainbow trout. These studies also indicated that heavy metals such as copper, zinc, and iron can accumulate within low and near-zero exchange WRAS without the use of ozone. In addition, ozone did not reduce dissolved potassium concentrations or nitrate nitrogen concentrations. The current research provided evidence that nitrate nitrogen could accumulate to very high levels (100–700 mg/L) within WRAS operated at low and near-zero exchange. Thus, WRAS operated at low and near-zero exchange could require denitrification unit processing (depending on exact feed loading and flushing rates) in order to avoid potentially toxic nitrate–nitrogen accumulation ( Van Rijn et al., 2006 ). The potential toxicity of each water quality parameter measured during these studies will be presented in detail within a companion paper ( Davidson et al., under review ). The flushing rates and subsequent feed loading rates that were used during the present studies represented the extreme or outer limit as compared to the recycle conditions that others have reported for fish culture, particularly for salmonids ( Table 7 ). Table 7 provides a comparison of cumulative feed burden (mg of daily feed/L of makeup water used daily) and feed loading rate (kg feed/m 3 makeup water/day) for a variety of systems with various flushing rates that have been used for fish culture. Note that the authors could not identify other literature that reported cumulative feed burdens nearly as high as those that were used during the present studies for salmonid culture. During Study 3, feed burden was as high as 1.47 × 10 5 mg daily feed/L daily makeup water within WRAS that used the lowest water exchange rates ( Table 7 ). The results from these studies suggest that the use of ozone enhances the culture tank water quality dramatically, thus increasing the feasibility of operating WRAS at extremely low water exchange rates that have not been previously attempted for the culture of a salmonid species such as a rainbow trout, which is relatively sensitive to deteriorating water quality conditions. Ultimately, these findings indicate an increased potential to locate fish culture facilities utilizing WRAS even in areas with very limited water resources, potentially near major cities with high demand for fresh seafood. Acknowledgements Special thanks to Karen Schroyer, Christine Marshall, Susan Glenn, and Susan Clements for water chemistry analysis; to Blake Cline and Kyle Crapster for assistance with fish husbandry and system maintenance; and to Kristin Kinman for assistance with statistical analysis. This research was supported by the USDA Agricultural Research Service under Agreement No. 59-1930-5-510. All experimental protocols and methods were in compliance with the Animal Welfare Act (9CFR) requirements and were approved by the Freshwater Institute's Institutional Animal Care and Use Committee. Use of trade names does not imply endorsement by the U.S. Government. References APHA, 2005 American Public Health Association (APHA) Standard Methods for the Examination of Water and Wastewater 21st ed. 2005 American Public Health Association Washington, DC Biesterfeld et al., 2003 S. Biesterfeld G. Farmer P. Russell L. Figueroa Effect of alkalinity type and concentration on nitrifying biofilm activity Water Environ. Res. 75 3 2003 196 204 Boyd, 2009 C.E. Boyd Trace metals toxic at high concentrations Global Aquacult. Advocate July/August 2009 24 26 Brazil, 1996 Brazil, B.L., 1996. Impact of ozonation on system performance and growth characteristics of hybrid striped bass ( Morone chrysops x M. saxatilis ) and tilapia hybrids ( Sarotherodon sp. ) reared in recirculating aquaculture systems. Ph.D. Dissertation, Virginia Polytechnic Institute and State University, Blacksburg, VA, USA. Bullock et al., 1997 G.L. Bullock S.T. Summerfelt A. Noble A.W. Weber M.D. Durant J.A. Hankins Ozonation of a recirculating rainbow trout culture system: I. Effects on bacterial gill disease and heterotrophic bacteria Aquaculture 158 1997 43 55 Chen et al., 1993 S. Chen M.B. Timmons D.J. Aneshansley J.H. Bisogni Suspended solids characteristics from recirculating aquacultural systems and design implications Aquaculture 112 1993 143 155 Chen et al., 2006 S. Chen J. Ling J. Blancheton Nitrification kinetics of biofilm as affected by water quality factors Aquacult. Eng. 34 2006 179 197 Christensen et al., 2000 J.M. Christensen K.A. Rusch R.F. Malone Development of a model for describing accumulation of color and subsequent destruction by ozone in a freshwater recirculating aquaculture system J. World Aquacult. Soc. 31 2000 167 174 Colt, 2006 J. Colt Water quality requirements for reuse systems Aquacult. Eng. 34 2006 143 156 Couturier et al., 2009 M. Couturier T. Trofimencoff J.U. Buil J. Conroy Solids removal at a recirculating salmon-smolt farm Aquacult. Eng. 41 2009 71 77 Davidson et al., 2009 J. Davidson C. Good C. Welsh B. Brazil S. Summerfelt Heavy metal and waste metabolite accumulation and their potential effect on rainbow trout performance in a replicated water reuse system operated at low or high flushing rates Aquacult. Eng. 41 2009 136 145 Davidson et al., under review Davidson, J., Good, C., Welsh, Summerfelt, S.T., under review. Increased deformities and unusual swimming in rainbow trout Oncorhynchus mykiss raised in low exchange water recirculation aquaculture systems . Aquacult. Eng. Deviller et al., 2005 G. Deviller O. Palluel C. Aliaume H. Asanthi W. Sanchez M.A. Franco Nava J.P. Blancheton C. Casellas Impact assessment of various rearing systems on fish health using multibiomarker response and metals accumulation Ecotoxicol. Environ. Safety 61 1 2005 89 97 EPA, 1986 U.S. Environmental Protection Agency, 1986. Quality Criteria for Water 1986. Publication EPA 440/5-86-001. USEPA, Office of Water Regulations and Standards, Washington, D.C. EPA, 1987 U.S. Environmental Protection Agency, 1987. Ambient water quality criteria for selenium—1987. Publication EPA 440/5-87-006. USEPA, Office of Water Regulations and Standards, Washington, D.C. EPA, 1996 U.S. Environmental Protection Agency, 1996. 1995 Updates: Water quality criteria for the protection of aquatic life in ambient water. Publication EPA-820-B-96-001. Washington, D.C. EPA, 2002 U.S. Environmental Protection Agency, 2002. National recommended water quality criteria. Publication EPA 822-R-02-047. USEPA, Office of Water, Washington, D.C. EPA, 2007 U.S. Environmental Protection Agency, 2007. Aquatic Life Ambient Freshwater Quality Criteria: Copper (2007 Revision). Publication EPA-822-R-07-001. USEPA, Office of Water, Washington, D.C. Fischer et al., 2009 G.J. Fischer J. Held C. Hartleb J. Malison Evaluation of brook trout production in a coldwater recycle aquaculture system Aquacult. Eng. 41 2009 109 113 Good et al., 2009 C. Good J. Davidson C. Welsh B. Brazil K. Snekvik S. Summerfelt The impact of water exchange rate on the health and performance of rainbow trout Oncorhynchus mykiss in water recirculation aquaculture systems Aquaculture 294 2009 80 85 Good et al., 2010 C. Good J. Davidson C. Welsh K. Snekvik S.T. Summerfelt The effects of carbon dioxide on performance and histopathology of rainbow trout Oncorhynchus mykiss in water recirculation aquaculture systems Aquacult. Eng. 42 2010 51 56 Grguric et al., 1994 G. Grguric J.H. Trefry J.J. Keaffaber Ozonation products of bromine and chlorine in seawater aquaria Water Res. 28 5 1994 1087 1094 HACH, 2003 Hach Company DR/4000 Spectrophotometer Procedures Manual 11th ed. 2003 Hach Company USA Heinen, 1996 J.M. Heinen E.M. Wade M.R. Jenkins Water quality criteria, uptake, bioaccumulation, and public health considerations for chemicals of possible concern in West Virginia mine waters used for culture of rainbow trout 1996 Conservation Fund's Freshwater Institute Shepherdstown, WV, USA Hozalski et al., 1995 R.M. Hozalski S. Goel E.J. Bouwer TOC removal in biological filters J. Am. Waste Water Assoc. 87 12 1995 40 54 Hutchinson et al., 1997 T.H. Hutchinson M.J. Hutchings K.W. Moore A review of the effects of bromate on aquatic organisms and toxicity of bromate to oyster ( Crassostrea gigas ) embryos Ecotoxicol. Environ. Safety 38 1997 238 243 Langlais et al., 1991 B. Langlais D.A. Recknow D.R. Brink Ozone in Water Treatment: Application and Engineering 1991 American Water Works Association Research Foundation and Lewis Publishers, Inc. Denver, CO, USA and Chelsea, MI, USA Loyless and Malone, 1997 C.L. Loyless R.F. Malone A sodium bicarbonate dosing methodology for pH management in freshwater-recirculating aquaculture systems Prog. Fish Cult. 59 1997 198 205 Maier, 1984 D. Maier R.G. Rice A. Netzer Handbook of ozone technology and applications 1984 Butterworth Boston, MA, USA pp. 123–140 Martins et al., 2009a C.I.M. Martins M.G. Pistrin S.S.W. Ende E.H. Eding J.A.J. Verreth The accumulation of substances in Recirculating Aquaculture Systems (RAS) affects embryonic and larval development in common carp, Cyprinus carpio Aquaculture 291 2009 65 73 Martins et al., 2009b C.I.M. Martins D. Ochola S.S.W. Ende E.H. Eding J.A.J. Verreth Is growth retardation present in Nile tilapia Oreochromis niloticus cultured in low water exchange recirculating aquaculture systems? Aquaculture 298 2009 43 50 Meade, 1989 J.W. Meade Aquaculture Management 1989 Van Nostrand Reinhold New York pp. 9 Morey, 2009 Morey, R.I., 2009. Design keys of a recent recirculating facility built in Chile operating with fluidized bed biofilters. Aquacult. Eng. 41, 85–90. Nerenberg et al., 2000 R. Nerenberg B.E. Rittman W.J. Soucie Ozone/biofiltration for removing MIB and geosmin J. Am. Water Works Assoc. 92 12 2000 85 95 Paller and Lewis, 1988 M.H. Paller W.M. Lewis Use of ozone and fluidized bed biofilters for increased ammonia removal and fish loading rates Prog. Fish Cult. 50 1988 141 147 Park et al., 2007 G. Park M. Yu J. Go E. Kim H. Kim Comparison between ozone and ferrate in oxidizing geosmin and 2-MIB in water Water Sci. Technol. 55 5 2007 117 125 Patterson and Watts, 2003 R.N. Patterson K.C. Watts Micro-particles in recirculating aquaculture systems: particle size analysis of culture water from a commercial Atlantic salmon site Aquacult. Eng. 28 2003 99 113 Patterson et al., 1999 R.N. Patterson K.C. Watts M.B. Timmons The power law in particle size analysis for aquacultural facilities Aquacult. Eng. 19 4 1999 259 273 Paz, 1984 Paz, J.D., 1984. The effects of borderline alkalinity on nitrification in natural water systems. Doctoral Dissertation. Polytechnic Institute of New York, New York. Piper et al., 1982 R.G. Piper I.B. McElwain L.E. Orme J.P. McCraren L.G. Fowler J.R. Leonard Fish Hatchery Management 1982 United States Department of the Interior Fish and Wildlife Service Washington D.C. Plummer and Edzwald, 2002 J.D. Plummer J.K. Edzwald Effects of chlorine and ozone on algal cell properties and removal of algae by coagulation J. Water Supply Res. T. 51 6 2002 307 318 Rice et al., 1981 R.G. Rice C.M. Robson G.W. Miller A.G. Hill Uses of ozone in drinking water treatment J. Am. Water Works Assoc. 73 1981 1 44 Ritar et al., 2006 A.J. Ritar G.G. Smith C.W. Thomas Ozonation of seawater improves the survival of larval southern rock lobster, Jasus edwardsii , in culture from egg to juvenile Aquaculture 261 3 2006 1014 1025 Roque d’Orbcastel et al., 2009a E. Roque d’Orbcastel J.P. Blancheton J. Aubin Towards environmentally sustainable aquaculture: comparison between two trout farming systems using life cycle assessment Aquacult. Eng. 40 2009 113 119 Roque d’Orbcastel et al., 2009b E. Roque d’Orbcastel Blancheton A. Belaud Water quality and rainbow trout performance in a Danish Model Farm recirculating system: comparison with flow through Aquacult Eng. 40 2009 135 143 Roque d’Orbcastel et al., 2009c E. Roque d’Orbcastel J.P. Ruyet N. Le Bayon J.P. Blancheton Comparative growth and welfare in rainbow trout reared in recirculating and flow through systems Aquacult. Eng. 40 2009 79 86 Rosenthal and Kruner, 1985 H. Rosenthal G. Kruner Treatment efficiency of an improved ozonation unit applied to fish culture situations Ozone-Sci. Eng. 7 1985 179 190 Rosenthal and Otte, 1980 H. Rosenthal G. Otte Ozonation in an intensive fish culture recycling system Ozone-Sci. Eng. 1 1980 319 327 Rueter and Johnson, 1995 J. Rueter R. Johnson The use of ozone to improve solids removal during disinfection Aquacult. Eng. 14 1995 123 141 Schrader et al., 2010 K.K. Schrader J. Davidson S.T. Summerfelt Evaluation of ozonation on levels of the off-flavor compounds geosmin and 2-methylisoborneol in water and rainbow trout Oncorhynchus mykiss from water recirculation aquaculture systems Aquacult. Eng. 43 2010 46 50 Sharrer and Summerfelt, 2007 M.J. Sharrer S.T. Summerfelt Ozonation followed by ultraviolet irradiation provides effective bacteria inactivation in a freshwater recirculating system Aquacult. Eng. 37 2007 180 191 Sigler et al., 1984 J.W. Sigler T.C. Bjorn F.H. Everest Effects of chronic turbidity on density and growth of steelheads and coho salmon Trans. Am. Fish. Soc. 113 1984 142 150 Skybakmoen et al., 2009 S. Skybakmoen S.I. Siikavuopio B.S. Saether Coldwater RAS in an Arctic char farm in Northern Norway Aquacult. Eng. 41 2009 114 121 Sokal and Rohlf, 1981 R.R. Sokal F.S. Rohlf Biometry 1981 W.H. Freeman New York Steslow, 1991 F.A. Steslow Ozone kinetics in seawater Freshwater Mar. Aquarium 14 1991 154 158 Suantika et al., 2001 G. Suantika P. Dhert G. Rombaut J. Vandenberghe T. DeWolf P. Sorgeloos The use of ozone in a high density recirculation system for rotifers Aquaculture 201 2001 35 49 Summerfelt, 2003 S.T. Summerfelt Ozonation and UV irradiation—an introduction and examples of current applications Aquacult. Eng. 28 2003 21 36 Summerfelt and Hochheimer, 1997 S.T. Summerfelt J.N. Hochheimer Review of ozone processes and applications as an oxidizing agent in aquaculture Prog. Fish Cult. 59 1997 94 105 Summerfelt et al., 1997 S.T. Summerfelt J.A. Hankins A.W. Weber M.D. Durant Ozonation of a recirculating rainbow trout culture system. II. Effects on microscreen filtration and water quality Aquaculture 158 1997 57 67 Summerfelt et al., 2004a S.T. Summerfelt M.J. Sharrer J. Hollis L.E. Gleason S.R. Summerfelt Dissolved ozone destruction using ultraviolet irradiation in a recirculating salmonid culture system Aquacult. Eng. 32 2004 209 224 Summerfelt et al., 2004b S.T. Summerfelt J. Davidson T. Waldrop S. Tsukuda J. Bebak-Williams A partial-reuse system for coldwater aquaculture Aquacult. Eng. 31 2004 157 181 Summerfelt et al., 2008 Summerfelt, S.T., Bebak-Williams, J., Fletcher, J., Carta, A., Creaser, D., 2008. Description of the surface water filtration and ozone treatment system at the Northeast Fishery Center. Pa in Amaral, S.V., Mathur, D., Taft III, E.P. (Eds.), Advances in Fisheries Bioengineering. American Fisheries Society, Symposium 61, Bethesda, MD, USA, pp. 97–121. Summerfelt et al., 2009a S.T. Summerfelt M.J. Sharrer S.M. Tsukuda M. Gearheart Process requirements for achieving full-flow disinfection of recirculating water using ozonation and UV irradiation Aquacult. Eng. 40 2009 17 27 Summerfelt et al., 2009b S.T. Summerfelt M. Sharrer M. Gearhart K. Gillette B.V. Vinci Evaluation of partial water reuse systems used for Atlantic salmon smolt production at White River National Fish Hatchery Aquacult. Eng. 41 2009 78 84 Sutterlin et al., 1984 A.M. Sutterlin C.Y. Courier T. Devereaux A recirculation system using ozone for the culture of Atlantic salmon Prog. Fish Cult. 46 1984 239 244 Tal et al., 2009 Y. Tal H.J. Schreier K.R. Sowers J.D. Stubblefield A.R. Place Y. Zohar Environmentally sustainable land-based marine aquaculture Aquaculture 286 2009 28 35 Tanaka and Matsumura, 2002 J. Tanaka M. Matsumura Kinetic studies of removal of ammonia from seawater by ozonation J. Chem. Technol. Biot. 77 2002 649 656 Tanaka and Matsumura, 2003 J. Tanaka M. Matsumura Application of ozone treatment for ammonia removal in spent brine Adv. Environ. Res. 7 2003 835 845 Tango and Gagnon, 2003 M.S. Tango G.A. Gagnon Impact of ozonation on water quality within marine recirculating systems Aquacult. Eng. 29 2003 125 137 Terishima, 1988 K. Terishima Reduction of musty odor substances in drinking water—a pilot plant study Water Sci. Technol. 20 8–9 1988 275 281 Van Rijn et al., 2006 J. Van Rijn Y. Tal H.J. Schreier Denitrification in recirculating systems: theory and applications Aquacult. Eng. 34 2006 364 376 Wedemeyer, 1996 G.A. Wedemeyer Physiology of Fish in Intensive Culture Systems 1996 Chapman and Hall New York Wolters et al., 2009 W. Wolters A. Masters B. Vinci S. Summerfelt Design, loading, and water quality in recirculating systems for Atlantic Salmon ( Salmo salar ) at the USDA ARS National Cold Water Marine Aquaculture Center (Franklin, Maine) Aquacult. Eng. 41 2009 60 70
MoreTranslated text
Key words
Ozone,Water reuse,Recirculating aquaculture system,Water quality,Copper,Heavy metals,Waste metabolite accumulation,Rainbow trout
AI Read Science
Must-Reading Tree
Example
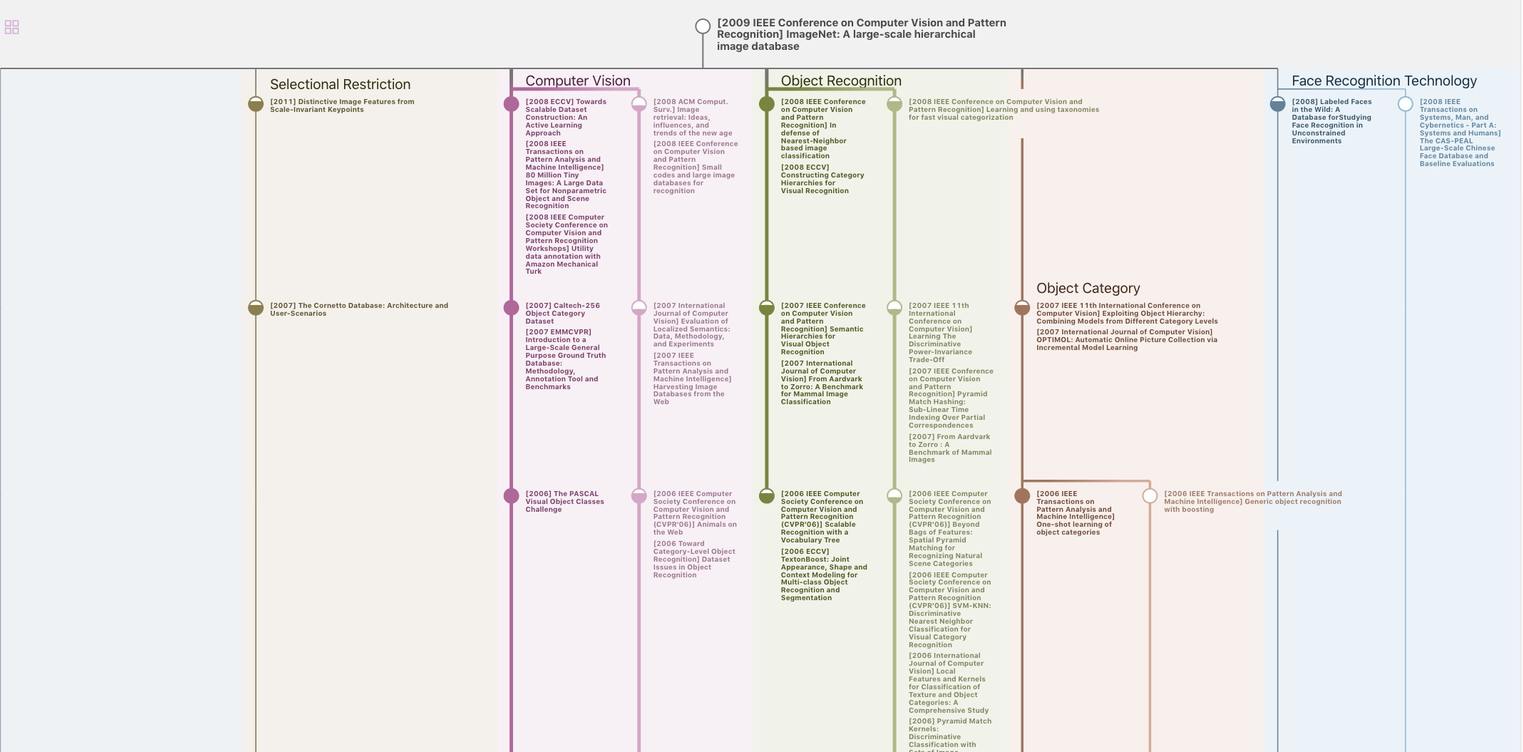
Generate MRT to find the research sequence of this paper
Chat Paper
Summary is being generated by the instructions you defined