The β3‐integrin endothelial adhesome regulates microtubule‐dependent cell migration
EMBO Reports(2018)
摘要
Scientific Report24 May 2018Open Access Transparent process The β3-integrin endothelial adhesome regulates microtubule-dependent cell migration Samuel J Atkinson Samuel J Atkinson School of Biological Sciences, Norwich Research Park, University of East Anglia, Norwich, UK Search for more papers by this author Aleksander M Gontarczyk Aleksander M Gontarczyk School of Biological Sciences, Norwich Research Park, University of East Anglia, Norwich, UK Search for more papers by this author Abdullah AA Alghamdi Abdullah AA Alghamdi School of Biological Sciences, Norwich Research Park, University of East Anglia, Norwich, UK Search for more papers by this author Tim S Ellison Tim S Ellison School of Biological Sciences, Norwich Research Park, University of East Anglia, Norwich, UK Search for more papers by this author Robert T Johnson Robert T Johnson School of Biological Sciences, Norwich Research Park, University of East Anglia, Norwich, UK Search for more papers by this author Wesley J Fowler Wesley J Fowler School of Biological Sciences, Norwich Research Park, University of East Anglia, Norwich, UK Search for more papers by this author Benjamin M Kirkup Benjamin M Kirkup School of Biological Sciences, Norwich Research Park, University of East Anglia, Norwich, UK Search for more papers by this author Bernardo C Silva Bernardo C Silva School of Biological Sciences, Norwich Research Park, University of East Anglia, Norwich, UK Search for more papers by this author Bronwen E Harry Bronwen E Harry School of Biological Sciences, Norwich Research Park, University of East Anglia, Norwich, UK Search for more papers by this author Jochen G Schneider Jochen G Schneider Luxembourg Center for Systems Biomedicine (LCSB), Luxembourg & Saarland University Medical Center, Internal Medicine II, University of Luxembourg, Homburg, Germany Centre Hospitalier Emily Mayrisch, Esch, Luxembourg Search for more papers by this author Katherine N Weilbaecher Katherine N Weilbaecher Division of Molecular Oncology, Department of Internal Medicine, Washington University in St Louis, St. Louis, MO, USA Search for more papers by this author Mette M Mogensen Mette M Mogensen School of Biological Sciences, Norwich Research Park, University of East Anglia, Norwich, UK Search for more papers by this author Mark D Bass Mark D Bass Department of Biomedical Science, Centre for Membrane Interactions and Dynamics, University of Sheffield, Sheffield, UK Search for more papers by this author Maddy Parsons Maddy Parsons orcid.org/0000-0002-2021-8379 Randall Division of Cell and Molecular Biophysics, King's College London, New Hunt's House, Guys Campus, London, UK Search for more papers by this author Dylan R Edwards Dylan R Edwards Faculty of Medicine and Health Sciences, Norwich Research Park, University of East Anglia, Norwich, UK Search for more papers by this author Stephen D Robinson Corresponding Author Stephen D Robinson [email protected] orcid.org/0000-0002-6606-7588 School of Biological Sciences, Norwich Research Park, University of East Anglia, Norwich, UK Search for more papers by this author Samuel J Atkinson Samuel J Atkinson School of Biological Sciences, Norwich Research Park, University of East Anglia, Norwich, UK Search for more papers by this author Aleksander M Gontarczyk Aleksander M Gontarczyk School of Biological Sciences, Norwich Research Park, University of East Anglia, Norwich, UK Search for more papers by this author Abdullah AA Alghamdi Abdullah AA Alghamdi School of Biological Sciences, Norwich Research Park, University of East Anglia, Norwich, UK Search for more papers by this author Tim S Ellison Tim S Ellison School of Biological Sciences, Norwich Research Park, University of East Anglia, Norwich, UK Search for more papers by this author Robert T Johnson Robert T Johnson School of Biological Sciences, Norwich Research Park, University of East Anglia, Norwich, UK Search for more papers by this author Wesley J Fowler Wesley J Fowler School of Biological Sciences, Norwich Research Park, University of East Anglia, Norwich, UK Search for more papers by this author Benjamin M Kirkup Benjamin M Kirkup School of Biological Sciences, Norwich Research Park, University of East Anglia, Norwich, UK Search for more papers by this author Bernardo C Silva Bernardo C Silva School of Biological Sciences, Norwich Research Park, University of East Anglia, Norwich, UK Search for more papers by this author Bronwen E Harry Bronwen E Harry School of Biological Sciences, Norwich Research Park, University of East Anglia, Norwich, UK Search for more papers by this author Jochen G Schneider Jochen G Schneider Luxembourg Center for Systems Biomedicine (LCSB), Luxembourg & Saarland University Medical Center, Internal Medicine II, University of Luxembourg, Homburg, Germany Centre Hospitalier Emily Mayrisch, Esch, Luxembourg Search for more papers by this author Katherine N Weilbaecher Katherine N Weilbaecher Division of Molecular Oncology, Department of Internal Medicine, Washington University in St Louis, St. Louis, MO, USA Search for more papers by this author Mette M Mogensen Mette M Mogensen School of Biological Sciences, Norwich Research Park, University of East Anglia, Norwich, UK Search for more papers by this author Mark D Bass Mark D Bass Department of Biomedical Science, Centre for Membrane Interactions and Dynamics, University of Sheffield, Sheffield, UK Search for more papers by this author Maddy Parsons Maddy Parsons orcid.org/0000-0002-2021-8379 Randall Division of Cell and Molecular Biophysics, King's College London, New Hunt's House, Guys Campus, London, UK Search for more papers by this author Dylan R Edwards Dylan R Edwards Faculty of Medicine and Health Sciences, Norwich Research Park, University of East Anglia, Norwich, UK Search for more papers by this author Stephen D Robinson Corresponding Author Stephen D Robinson [email protected] orcid.org/0000-0002-6606-7588 School of Biological Sciences, Norwich Research Park, University of East Anglia, Norwich, UK Search for more papers by this author Author Information Samuel J Atkinson1, Aleksander M Gontarczyk1, Abdullah AA Alghamdi1, Tim S Ellison1, Robert T Johnson1, Wesley J Fowler1, Benjamin M Kirkup1, Bernardo C Silva1, Bronwen E Harry1, Jochen G Schneider2,3, Katherine N Weilbaecher4, Mette M Mogensen1, Mark D Bass5, Maddy Parsons6, Dylan R Edwards7 and Stephen D Robinson *,1 1School of Biological Sciences, Norwich Research Park, University of East Anglia, Norwich, UK 2Luxembourg Center for Systems Biomedicine (LCSB), Luxembourg & Saarland University Medical Center, Internal Medicine II, University of Luxembourg, Homburg, Germany 3Centre Hospitalier Emily Mayrisch, Esch, Luxembourg 4Division of Molecular Oncology, Department of Internal Medicine, Washington University in St Louis, St. Louis, MO, USA 5Department of Biomedical Science, Centre for Membrane Interactions and Dynamics, University of Sheffield, Sheffield, UK 6Randall Division of Cell and Molecular Biophysics, King's College London, New Hunt's House, Guys Campus, London, UK 7Faculty of Medicine and Health Sciences, Norwich Research Park, University of East Anglia, Norwich, UK *Corresponding author. Tel: +44 1603 591756; E-mail: [email protected] EMBO Reports (2018)19:e44578https://doi.org/10.15252/embr.201744578 PDFDownload PDF of article text and main figures. Peer ReviewDownload a summary of the editorial decision process including editorial decision letters, reviewer comments and author responses to feedback. ToolsAdd to favoritesDownload CitationsTrack CitationsPermissions ShareFacebookTwitterLinked InMendeleyWechatReddit Figures & Info Abstract Integrin β3 is seen as a key anti-angiogenic target for cancer treatment due to its expression on neovasculature, but the role it plays in the process is complex; whether it is pro- or anti-angiogenic depends on the context in which it is expressed. To understand precisely β3's role in regulating integrin adhesion complexes in endothelial cells, we characterised, by mass spectrometry, the β3-dependent adhesome. We show that depletion of β3-integrin in this cell type leads to changes in microtubule behaviour that control cell migration. β3-integrin regulates microtubule stability in endothelial cells through Rcc2/Anxa2-driven control of active Rac1 localisation. Our findings reveal that angiogenic processes, both in vitro and in vivo, are more sensitive to microtubule targeting agents when β3-integrin levels are reduced. Synopsis Engagement of αvβ3-integrin with fibronectin at mature focal adhesions localises an Rcc2/Anxa2/Rac1 containing complex to these sites, preventing Rac1 from stabilising microtubules. When αvβ3 is not present, the complex associates with α5β1-integrin instead, resulting in increased microtubule stability. β3-integrin regulates localisation of tubulin subunits to the endothelial adhesome. Angiogenic processes both in vitro and in vivo, are more sensitive to microtubule targeting agents when β3-integrin levels are reduced. Active Rac1 cellular associations change with depletion of β3-integrin. Introduction Angiogenesis, the formation of new blood vessels from those that already exist, plays an essential role in tumour growth 1. As such, targeting angiogenesis is seen as crucial in many anti-cancer strategies 2. Therapies directed against vascular endothelial growth factor (VEGF) and its major receptor, VEGF-receptor-2 (VEGFR2), whilst effective in a number of cancers, are not without side-effects due to the role this signalling pathway plays in vascular homeostasis 3. Fibronectin (FN)-binding endothelial integrins, especially αvβ3- and α5β1-integrins, have emerged as alternative anti-angiogenic targets because of their expression in neovasculature 4, 5. However, neither global nor conditional knockouts of these integrins block tumour angiogenesis in the long term 6-8, and clinical trials of blocking antibodies and peptides directed against these extracellular matrix (ECM) receptors have been disappointing 9, 10. To gain novel insight into how αvβ3-integrin regulates outside-in signal transmission 11, we have undertaken an unbiased analysis of the molecular composition of the mature endothelial adhesome and profiled changes that occur when β3-integrin expression is manipulated. In so doing, we have uncovered β3-integrin-dependent changes in microtubule behaviour that regulate cell migration. Results and Discussion The isolation and analysis of integrin adhesion complexes (IACs) by mass spectrometry (MS) is difficult because of the low affinity and transient nature of the molecular interactions occurring at these sites. However, using cell-permeant chemical cross-linkers improves recovery of IAC proteins bound to either FN-coated microbeads 12 or plastic dishes 13. These advances have led to the characterisation of IACs from a number of cell types. Whilst a core consensus adhesome (the network of structural and signalling proteins involved in regulating cell-matrix adhesion 14) can be defined 12, the composition and stoichiometry of the meta-adhesome depend on the cell type being analysed, the integrin-receptor repertoire expressed by that cell type, and any imposed experimental conditions. To examine the composition of the endothelial adhesome, we isolated lung microvascular endothelial cells (ECs) from C57BL6/129Sv mixed background mice and immortalised them with polyoma-middle-T-antigen by retroviral transduction 15. As our main interest was in establishing how β3-integrin influences the endothelial adhesome, we adhered cells to FN for 90 min, which allows β3-rich (mature) focal adhesions (FAs) to form 16. To distinguish integrin-mediated recruitment of proteins from non-specific background, we also plated cells on poly-l-lysine (PLL) as a negative control (adhesion to PLL does not depend on integrins). Visualisation of neuropilin-1 staining in whole cells showed that this protein, which we previously demonstrated is present in the mature EC adhesome 17, co-localises with talin-1 in FAs when cells are plated on FN, but not PLL (Fig 1A). For all proteomics experiments, we cross-linked FAs using the cell-permeant and reversible cross-linkers DPDPB and DSP (see Materials and Methods) for 5 min. Cells were lysed and subjected to a high shear flow water wash to remove non-cross-linked material. Crosslinking was reversed, and samples were precipitated and concentrated for analyses. Prior to MS, samples were quality-controlled by SDS–PAGE and silver staining to ensure efficient removal of non-cross-linked material had occurred (Fig 1B). Figure 1. Defining the FN endothelial adhesome WT ECs were adhered to fibronectin- (top row) or poly-l-lysine (bottom row)-coated coverslips for 90 min before fixing and immunostaining for neuropilin-1 (Nrp1, green) and talin-1 (Tln1, red) along with a nuclear stain (DAPI, blue). Scale bar = 10 μm. An example silver stain used in the quality control of adhesome samples. Adhesome enrichment was carried out on 6 × 106 WT ECs on fibronectin (FN), fibronectin with VEGF (FN + VEGF) or poly-l-lysine (PLL) before acetone precipitation. After resuspension, samples were run on a SDS–PAGE gel along with a whole cell lysate control and silver-stained. Triplicate adhesome samples from WT ECs adhered on fibronectin (FN), fibronectin with VEGF (FN + VEGF) or poly-l-lysine were sent for quantitative mass spec analysis. Label-free quantification was carried out using MaxQuant followed by analysis in Perseus. Unsupervised hierarchical clustering (Euclidian distance calculation) was carried out with red showing highly abundant proteins and green showing low abundance proteins. Twelve significant clusters were automatically identified using a distance threshold of 3.34 and labelled as A–L. Angiogenesis-associated proteins were identified using GOBP annotations (GO:0001525, GO:0002040, GO:0002042, GO:0016525, GO:0045765, GO:0045766) and are displayed in the table along with their associated cluster. Download figure Download PowerPoint Label-free proteomic analyses of the FN + VEGF, FN, and PLL adhesomes (Fig 1C; Table EV1) initially detected and quantified 1,468 proteins. Stringent filtering, requiring proteins to be detected in all three repeats of at least one condition, left 1,064 proteins—a high confidence dataset that was used to define the endothelial adhesome. Hierarchical clustering based on average Euclidian distance identified 12 clusters (A–L), which could be considered VEGF-enriched (A–C), FN-enriched (D–F) and PLL-enriched (G–L) proteins. Fisher's exact test enrichment analysis was carried out to identify which pathway, process or component proteins within these clusters belong to using Gene Ontology annotations. Cell projection (GOCC, P = 8.62 × 10−5) and microtubule (GOCC, P = 1.6 × 10−4) categories were significantly enriched when cells were treated with growth factor, suggesting they are important in VEGF-mediated processes. Leucocyte trans-endothelial migration (KEGG, P = 9.71 × 10−5) proteins were enriched in the FN adhesome, but not in the VEGF-stimulated adhesome, suggesting our cells represent quiescent vasculature without VEGF stimulation. This same category contains many endothelial-specific proteins (e.g. VE-cadherin, Cdh5), further confirming that the cells have an endothelial identity. Focal adhesion (KEGG, P = 9.31 × 10−7) proteins were enriched in the FN adhesome but depleted in the PLL adhesome, confirming the success of the adhesome enrichment process, MS and downstream analysis. Other adhesion/migration-associated categories, focal adhesion (GOCC, P = 5.99 × 10−5), cell projection (GOCC, P = 3.03 × 10−5), cell adhesion (GOBP, P = 1.61 × 10−6) and lamellipodium (GOCC, P = 1.38 × 10−4), were depleted in the PLL adhesome. To test the consequences of excluding β3-integrin from the EC adhesome, we decided to profile changes in β3-heterozygous (β3HET) ECs, which carry one wild-type allele of β3-integrin and one knockout allele. These cells express 50% wild-type levels of β3-integrin. As in previous studies, we decided to use β3HET cells for these initial analyses, rather than β3-integrin knockout (β3NULL) cells, hypothesising this would circumvent potential developmental changes arising from the complete loss of the protein, which we felt might confound quantitative interpretations of the EC adhesome; we have shown these cells are a good model for studying the role of αvβ3-integrin in cell migration, whilst evading changes arising from the complete loss of the integrin on both alleles (e.g. up-regulated total VEGFR2 expression) 17. Both wild-type (β3WT) and β3HET ECs adhere equally to saturating concentrations (10 μg ml−1) of FN (see Ellison et al 17). To compare the size distribution of FAs between β3WT and β3HET ECs (which might affect the stoichiometry of components in the adhesome), we seeded cells for 90 min on FN, immunostained for paxillin, and measured FA area; we noted no differences in the percentage of FA size distributions between the two genotypes (Fig 2A). Therefore, MS analyses comparing the adhesome between β3WT and β3HET ECs were performed (Fig 2B; Table EV2). Enrichment analysis showed a depletion of cytoskeletal components (GOCC, P = 4.73 × 10−5) in the β3WT adhesome when compared with the β3HET adhesome, despite the enrichment of adhesion/migration-associated categories previously noted in the FN adhesome of β3WT ECs (Fig 1C). Whilst a majority of individual FA components in the mature adhesome do not change upon β3-integrin depletion, downstream connections to cytoskeletal components do. We took a particular interest in microtubules (MTs) because by SAM analysis all detected tubulins were significantly up-regulated in the β3HET adhesome. To confirm this finding by other means, we probed Western blots for α-tubulin and showed a significant increase in FA-enriched samples from β3HET cells compared with β3WT cells (Fig 2C). Figure 2. Analysis of the β3-integrin-dependent adhesome Distribution of adhesion size classes (0–2 μm; 2–10 μm; > 10 μm) in β3WT versus β3HET endothelial cells (n = 1,400 FAs per genotype, from two independent experiments). Visual representation of the significance analysis of microarrays (SAM) method as a volcano plot for β3WT and β3HET samples (n = 3). t-Test difference is plotted against −log of the P-value. The blue lines show the cut-off for significance as defined by the SAM. Integrin-β3 (Itgb3) as well as all detected tubulins (Tub) have been highlighted as red points. Adhesome samples from β3WT and β3HET endothelial cells adhered to fibronectin. Samples were Western-blotted for integrin-β3 (Itgb3), α-tubulin and heat-shock protein 70 (Hspa1a). Blot shown is representative of the five individual experiments that are quantified in the bar graph below. Bars = mean (±SEM) relative α-tubulin levels normalised to Hspa1a levels. Significant differences between means were evaluated by unpaired two-tailed Student's t-test. Download figure Download PowerPoint Our findings intimated that αvβ3-integrin drives MT localisation away from FAs. To increase the power of our downstream mechanistic analyses, we felt it was appropriate to now also include β3NULL ECs in our studies. We examined MT organisation in β3WT, β3HET and β3NULL ECs by immunolabelling for α-tubulin in whole cells (Fig 3A). No gross changes in cell microtubule arrays were observed. Furthermore, total cellular levels of α-tubulin were similar across all three genotypes (Fig 3B). However, co-localisation of MTs at peripheral FAs was greater in β3HET and β3NULL ECs, compared to β3WT ECs, as was extension into lamellipodia (Fig 3C; for an example of quantification of the latter, see Fig EV1). Overall, the findings suggest that β3-integrin limits the targeting of MTs to FAs. Figure 3. Analysis of microtubules in β3WT, β3HET and β3NULL endothelial cells β3WT, β3HET and β3NULL endothelial cells were adhered to fibronectin-coated coverslips for 90 min before being PHEMO fixed and immunostained for α-tubulin (green). Nuclear (DAPI, blue) and phalloidin (F-actin, red) stains were also used. Inverted black and white images of α-tubulin and F-actin are shown below the three-colour overlays. Scale bar = 10 μm. β3WT, β3HET and β3NULL endothelial cells were adhered to fibronectin for 90 min before being lysed and Western-blotted for integrin-β3 (Itgb3), α-tubulin and Gapdh (as a loading control). Left: β3WT, β3HET and β3NULL endothelial cells were adhered to fibronectin-coated coverslips for 90 min before being methanol (−20°C) fixed and immunostained for α-tubulin and talin-1. The number of microtubules that terminated (overlapping staining) at a talin-1 containing focal adhesion was counted for each genotype (n = 15 cells per genotype, from three independent experiments). Right: β3 WT, HET and NULL ECS were transfected with paxillin-GFP and left to recover overnight. The cells were then adhered to fibronectin-coated coverslips and allowed to recover for 3 h before being treated with 100 nM SiRTubulin and 1 μM verapamil overnight. The next day, fresh media containing SiRTubulin and verapamil (same dose) were added and cells were imaged every minute for 30 min (n = 3 cells per genotype, from three independent experiments). Areas of adhesive fronts were assessed by measuring the growth of paxillin–GFP-positive areas between the 1st and 30th image. The number of microtubules that entered the adhesive front was quantified to give the number of microtubules entering lamellipodia relative to the area of adhesive fronts for each cell. Significant differences between means were evaluated by unpaired two-tailed Student's t-test. Error bars are ±SEM. β3WT, β3HET and β3NULL endothelial cells were adhered to fibronectin overnight. Migration speed of individual cells was measured over 15 h using the MTrackJ plugin for ImageJ under the influence of the indicated MTA. Migration speeds are shown as a percentage of the speed of the corresponding genotype under DMSO (vehicle) treatment (n ≥ 46 cells per genotype, from four independent experiments). Significant differences between means were evaluated by unpaired two-tailed Student's t-test. Error bars are ±SEM. β3flox/flox Tie1Cre-positive (pos) and Cre-negative (neg) animals were injected subcutaneously with 1 × 106 CMT19T lung carcinoma cells and then treated with vehicle (veh), or Eribulin (Eri). Bar graph shows mean (±SEM) tumour volumes (n ≥ 6; from two to three independent experiments for each treatment condition) at the end of the experiment. Micrographs (below) show representative tumours. Scale bars = 5 mm. After excision, tumours from β3flox/flox Tie1Cre-positive (pos) and Cre-negative (neg) animals were processed and CD31 staining was assessed in vessel hot spots (see Materials and Methods) to measure vascular density. Bars = mean (±SEM) vessel number per mm2 (n = 5 sections from each genotype, taken over two to three independent experiments for each treatment condition). Micrographs (below) show representative images of sections stained for alpha-smooth muscle actin (αSMA, green), CD31 (red), DAPI (blue). Dotted white line indicates border of tumour and surrounding connective tissue. Scale bars = 100 μm. Download figure Download PowerPoint Click here to expand this figure. Figure EV1. Measuring microtubule extension into lamellipodiumSchematic demonstrating how Fig 3C (right) was calculated. The yellow line indicates the edge of Pxn-GFP (green)-positive areas at 0 min, and the blue line indicates the edge at the end of 30 min. Microtubules were labelled red with SiRTubulin. Scale bar = 5 μm. Download figure Download PowerPoint Given that MTs can drive FA turnover and thus cell migration 18, we next tested whether EC migration is differentially sensitive to microtubule targeting agents (MTAs) in β3HET and β3NULL ECs. For each MTA examined, we first determined the dose of the compound that allowed 90% survival of β3WT ECs (see Materials and Methods) and then tested the effects of this dose on random migration in β3WT, β3HET and β3NULL cells (Fig 3D; raw migration data shown in Fig EV2). Random migration was affected by MT stabilisers (Paclitaxel, Epothilone B) in cells of all three genotypes, However, β3WT ECs were insensitive to the MT destabilisers tested (Colchicine, Mebendazole, Fosbretabulin) and the mechanistically unique MTA Eribulin (which functions through an end poisoning mechanism 19), whilst β3HET and β3NULL ECs generally showed a sensitivity to all classes of compounds tested. We extended these types of analyses in vivo to examine the effects of Eribulin and Fosbretabulin on tumour growth and angiogenesis. We chose these two MTAs as they are well tolerated in mice 20, 21 and used clinically in humans. We settled on suboptimal doses (see Materials and Methods) that would allow us to observe potential synergy with endothelial depletion of β3-integrin. β3-integrin-floxed/floxed mice 22 were bred with Tie1Cre mice 23 to generate β3-integrin-floxed/floxed Cre-positive animals (Cre-negative littermates were used as controls). CMT19T lung carcinoma cells were injected subcutaneously and allowed to establish for 7 days, at which point the MTAs were administered (see Materials and Methods for dosing regimes). Neither Eribulin nor Fosbretabulin had any effect on tumour growth in Cre-negative animals compared with vehicle-treated animals, but tumour growth was reduced in MTA-treated Cre-positive animals (Fig 3E). Analysis of tumours by immunostaining for blood vessels showed a reduction in intratumoral microvascular density only in sections from MTA-treated Cre-positive animals (Fig 3F). These studies suggested that the loss of endothelial β3-integrin sensitises angiogenic responses to MT destabilisers both in vitro and in vivo. Click here to expand this figure. Figure EV2. Effects of MTAs on EC migration speedsβ3WT, β3HET and β3NULL endothelial cells were adhered to fibronectin overnight. Migration speed of individual cells was measured over 15 h using the MTrackJ plugin for ImageJ under the influence of the indicated MTA. Bars = mean migration speed (±SEM) (n ≥ 46 cells per genotype, from four independent experiments). Significant differences between means were evaluated by unpaired two-tailed Student's t-test. Download figure Download PowerPoint The increased sensitivity to destabilising MTAs suggested to us that there is an increased population of stable MTs in β3HET and β3NULL ECs compared with their wild-type counterparts. We explored this premise by exposing ECs to cold temperatures (which destabilises MTs), washing out tubulin monomers 24, followed by immunolabelling for α-tubulin. We noted elevated stable MTs in both β3HET and β3NULL cells (Fig 4A). Re-introducing β3-integrin into β3NULL cells restored MT sensitivity to cold; MTs were more sensitive to cold treatment in NULL cells expressing full-length human β3-integrin, than MTs in cells transfected with an empty vector control (Fig EV3). We also measured MT stability biochemically by extracting both cold-sensitive and cold-stable MTs from the same sample of cold-treated cells and Western blotting for α-tubulin (Fig 4B). On whole, β3HET and β3NULL ECs showed decreased cold-sensitive and increased cold-stable MTs compared with β3WT ECs. Figure 4. Analysis of microtubule stability in β3WT, β3HET and β3NULL endothelial cells Top: β3WT, β3HET and β3NULL endothelial cells were adhered to fibronectin-coated coverslips for 75 min at 37°C before being moved to ice for 15 min. Soluble tubulin was then washed out using PEM buffer (see Materials and Methods) before fixing with −20°C methanol (Note: this protocol leads to nuclear auto-fluorescent background in all three channels used). Immunostaining was carried out for α-tubulin (green) and talin-1 (Tln1, red). DAPI (blue) was used as a nuclear stain. Images shown are representative of the data shown in the bar graph shown below. Scale bar = 5 μm. Bottom: Dot plots = mean (±SEM) number of cold-stable microtubules per cell (n ≥ 300 cells per genotype, from three independent experiments). Significant differences between means were evaluated by unpaired two-tailed Student's t-test. β3WT, β3HET and β3NULL endothelial cells were adhered to fibronectin for 75 min at 37°C before being moved to ice for 15 min. Cold-soluble tubulin (left blot) was then washed out using PEM buffer and Western-blotted for α-tubulin and Gapdh (as a loading control). Cold-insoluble tubulin (middle blot) from the same cells was obtained by lysing the remaining cells and Western blotting for α-tubulin and Gapdh (as a loading control). Right bar chart: Bars = mean (±SEM) relative cold-soluble and cold-insoluble α-tubulin levels for each genotype. Data are representative of four independent experiments. *indicates statistical significance compared to WT (P < 0.05). Significant differences between means were evaluated by unpaired two-tailed Student's t-test. Download figure Download PowerPoint Click here to expand this figure. Figure EV3. Measuring the effects on microtubule stability of reintroducing β3-integrin into β3NULL ECsTop: β3NULL endothelial cells were transfected with a full-length human β3-integrin (hβ3) cDNA expression construct or an empty vector (EV) control and Western-blotted for β3-integrin (β3NULL parent cells shown for comparison). Bottom: β3NULL + EV or β3NULL + hβ3 endothelial cells were adhered to fibronectin-coated coverslips for 75 min at 37°C before being moved to ice for 15 min. Soluble tubulin was then washed out using PEM buffer (see Materials and Methods) before fixing with −20°C methanol. Immunostaining was carried out for α-tubulin (green) and talin-1 (Tln1-red). DAPI (blue) was used as a nuclear
更多查看译文
关键词
endothelial,adhesome regulates,cell
AI 理解论文
溯源树
样例
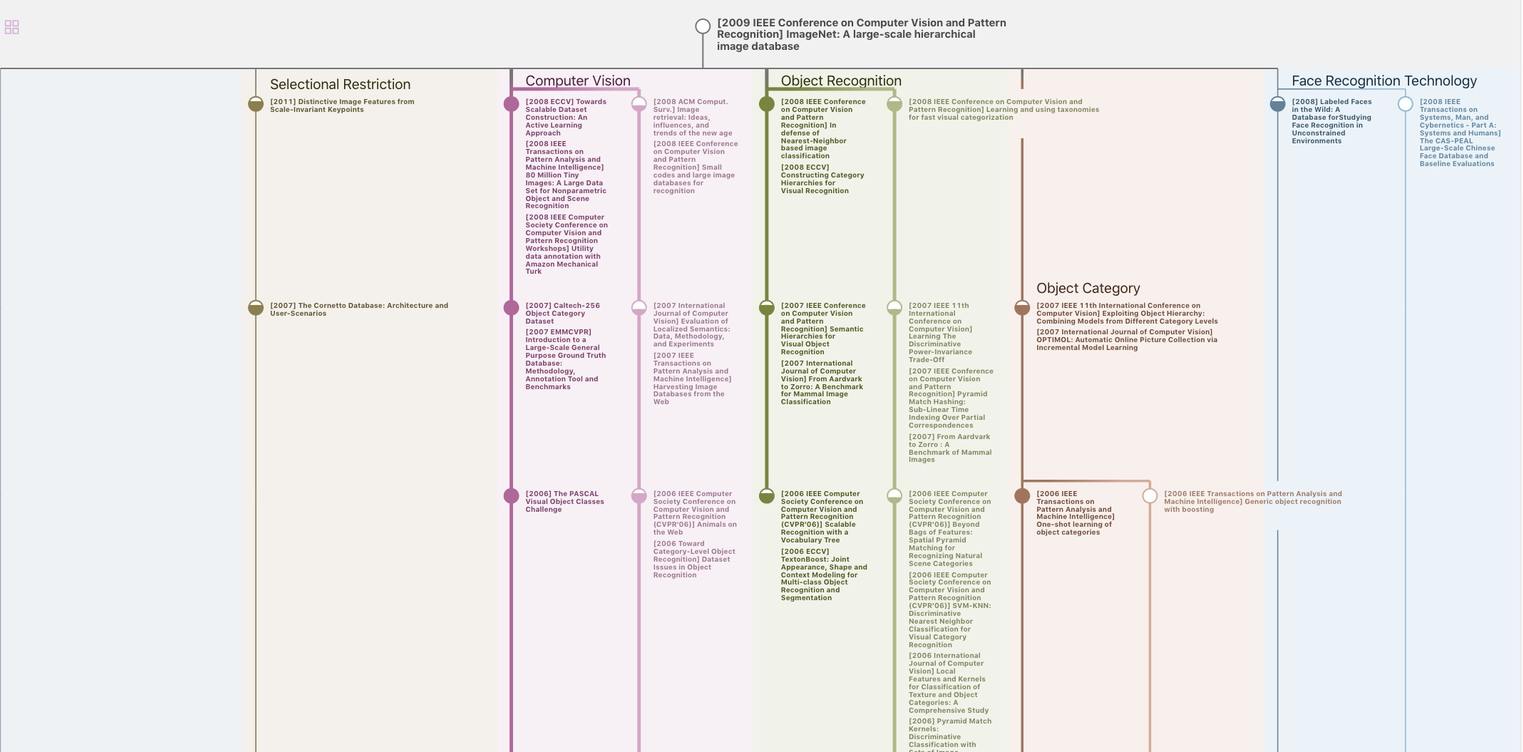
生成溯源树,研究论文发展脉络
Chat Paper
正在生成论文摘要