Detecting long‐term changes in stomatal conductance: challenges and opportunities of tree‐ring δ 18 O proxy
New Phytologist(2022)
摘要
There is growing scientific consensus that rising atmospheric CO2 concentrations have caused an increase in forest water-use efficiency (WUE), but the magnitude of this effect and the relative roles of enhanced photosynthesis vs reduced stomatal conductance (gs) are still under debate (Walker et al., 2020). In Guerrieri et al. (2019) we addressed this by combining 30 years of tree-ring stable carbon (δ13C) and oxygen (δ18O) isotope data with a water–carbon optimality model for 12 tree species at eight sites within the AmeriFlux network in the US. The results suggested that enhanced photosynthesis has been widespread, while reduced gs was more common at sites experiencing frequent water limitation. In this issue of New Phytologist, Lin et al. (2022) challenges these findings based on three criticisms pertaining to the use and interpretation of δ18O data. While the physiological interpretation of δ18O in tree rings is indeed complex, it remains the only proxy – in conjunction with δ13C – that can be used to reconstruct mechanisms through which WUE changes in response to global change drivers, including atmospheric CO2. Here, we address the criticisms raised by Lin et al. (2022), with the aim of moving the discussion forward and hence encouraging the use of tree-ring δ18O as a proxy to constrain plant and forest ecophysiology and the response to environmental changes beyond climate variability. Criticism 1: Lin et al. (2022) questioned the approach employed in Guerrieri et al. (2019) to estimate temporal changes in δ18O in precipitation (δ18OP), which relies on equations presented in Barbour et al. (2001) (‘B01 model’ hereafter) to assess spatial δ18OP. In particular, Guerrieri et al. (2019) used temperature and precipitation (both as annual or growing season means) and elevation as predictors. They considered eight sites across North America (most of the sites were in Canada, and thus did not overlap with the sites studied in Guerrieri et al., 2019) where δ18OP values were available from the global network of isotopes in precipitation (GNIP) for the same time window we considered in Guerrieri et al. (2019). The nonsignificant relationship between estimated and measured δ18OP found at those sites led the authors to conclude that the B01 model may not be adequate for the estimation of the isotopic signature of precipitation, and that this would, therefore, likely be the case for our sites. We acknowledge that the time for space substitution of the B01 model bears uncertainties that can limit the interpretation of our results. Yet, in the absence of δ18OP observations, such an approach is valuable, and we applied it carefully, and in the limited context of our study sites: inferring the directionality of the trend in the transpiration (E)–gs relationship. In Guerrieri et al. (2019), the δ18OP estimates were validated with 2 years of δ18O measurements in soil water (δ18Osw) at two sites with contrasting climates, with the assumption that δ18Osw will reflect δ18OP. As shown in supporting information fig. S11 (Guerrieri et al., 2019), the estimates of δ18OP were in good agreement with measured δ18Osw, particularly at the site in Florida, where precipitation patterns may be more complex compared to those of other northeastern US sites, due to the humid subtropical climate. Moreover, we know that measurements made in the first 10 cm of the soil may not accurately reflect δ18OP due to the effect of evaporation, thus leading to a deviation between modelled δ18OP and measured δ18Osw; but this did not seem to particularly affect the site in Florida, which had sandy soil and a higher air temperature than the Harvard Forest in northeastern US. The B01 model may not provide a robust estimate of the absolute or interannual variability of δ18OP values; however, temperature and precipitation remain two of the most important factors that affect δ18OP, and these factors provide reliable predictions of global δ18OP such as those used in isoscapes (Bowen, 2010). More sophisticated approaches were recently proposed to estimate spatial and temporal changes in precipitation δ18O (Terzer et al., 2013; Allen et al., 2018 at global scale; Nelson et al., 2021 for Europe), which will greatly contribute to reducing the uncertainties in source water δ18O estimates. When δ18OP data are not available, estimating δ18OP variations following the B01 model remains a valuable and more simple approach to accounting for the variation in source water δ18O in the temporal trend of tree ring δ18O, and to improving the detection of tree physiological signals, when the focus is the long-term pattern (rather than intra-annual) reconstruction of source water δ18O. At most of the sites considered by Lin et al. (2022) (see their fig. 1), the estimate from the B01 model and the observed δ18OP values showed a consistent directionality of trend (given the positive Pearson coefficient for all but two sites), despite the statistical significance of the correlations. Indeed, values from GNIP usually derive from monthly precipitation water (including snow) collection and therefore have a high temporal resolution which can substantially differ from (smoothed) estimates based on integrated periods such as mean growing season or mean annual temperature and precipitation. A critical point regarding δ18OP concerns the different water sources trees can access, that is, different origins (seasonal) and soil depths (Brinkmann et al., 2018; Allen et al., 2019; Goldsmith et al., 2022). We addressed this, though only at two sites (with contrasting climates) where data on soil and xylem water δ18O were available (fig. S9 in Guerrieri et al., 2019). The estimated δ18OP fell within the confidence interval of both measured soil and xylem δ18O values. This is aligned with other observations (depending on site, soil type, rooting depth and tree species) that xylem water is similar to soil water (seldom the top soil δ18O, e.g. Treydte et al., 2014). The uncertainties in the leaf water 18O enrichment (Δ18OLW) estimates that Lin et al. (2022) attributed to the estimate of δ18OP (obtained from the B01 model, fig. S9 in Guerrieri et al., 2019) could be related to the fact that measurements for given days were compared with estimates integrated over the growing season. The absolute values of estimated and measured Δ18OLW are not expected to match, given that the measured Δ18OLW is based on sample collections from 2–3 d and from two trees (as detailed in the supporting information for Guerrieri et al., 2019). Despite that, our estimates fell within the confidence interval of the measured Δ18OLW, which gives us confidence that our estimates could capture the temporal dynamics of leaf water enrichment. Criticism 2: The second point raised by Lin et al. (2022) was related to the interpretation of Δ18OLW (as calculated from tree-ring δ18O and estimated δ18OP) in terms of qualitative changes in gs, and underlying assumptions regarding the Péclet effect. Our gs–Δ18OLW interpretation was not carried out a priori, but in relation to changes in moisture conditions (vapour pressure deficit (VPD), relative humidity (RH), precipitation, standardised precipitation–evapotranspiration index (SPEI)) and evapotranspiration (ET) as derived from eddy covariance data (fig. S15 in Guerrieri et al., 2019). We also considered differences in physiological strategies between plant functional type, physiological metrics derived from δ13C (intercellular CO2 concentrations, carbon isotope discrimination) and basal area increment. That is, we used the relationships found in previous studies (Barbour et al., 2000; Sullivan & Welker, 2006; Grams et al., 2007) in a conceptual framework in which environmental and physiological metrics were considered to constrain the physiological interpretation of the estimated Δ18OLW in terms of gs (Siegwolf et al., 2021). Interpreting the gs–Δ18OLW relationship as a function of changes in VPD is physiologically plausible, given the well-established relationship between gs and VPD (Grossiord et al., 2020). Under more xeric conditions, gs is expected to decrease with increasing VPD (Grossiord et al., 2020), which is reflected in a reduced dilution of the enriched (in 18O) leaf water with nonenriched xylem water at lower transpiration rates at high VPD (Cernusak et al., 2016); thus, the Péclet effect has a smaller influence on the predicted leaf water δ18O enrichment when compared to measured δ18O values (Belmecheri et al., 2018). Under mesic conditions and with no temporal changes in precipitation, RH, VPD or SPEI, it is physiologically plausible to assume that gs remains unchanged, given that ET at those sites has not changed (fig. S15 in Guerrieri et al., 2019). Other studies considering mesic sites in the northeastern USA showed that with the climate becoming wetter, stomatal limitations on photosynthesis are reduced, despite increasing atmospheric CO2 concentrations (Levesque et al., 2017; Belmecheri et al., 2021, the latter including also some of the sites in Guerrieri et al., 2019). The finding that gs did not decrease over recent decades is in line with other findings based on different data and approaches (e.g. Long et al., 2004; Paschalis et al., 2017; Purcell et al., 2018; Cernusak et al., 2019). The calculation of Δ18OLW from tree-ring δ18O in Guerrieri et al. (2019) did not account for changes in the absolute values of the effective path length for water movement through the leaf (L factor). The latter may show hourly variations in relation to changes in RH, and those changes followed a similar pattern across a number of days, but the absolute values measured on different days remained within the same range (see, for instance, fig. 1 in Song et al., 2013). Diurnal variations in leaf water 18O strongly reflect changes in weather conditions, which can be smoothed in tree-ring δ18O, as the latter records a signal integrated over the growing season (several weeks/months) and in response to climate variations. Most of the studies quantifying values of L have been on seedlings and on a few selected days (e.g. Song et al., 2013), and these conditions may not be representative of the conditions that mature trees experience throughout the growing season. Nevertheless, if we use the ‘modelling exercise’ presented in fig. 2 by Lin et al. (2022), given that ET, RH and precipitation across our mesic sites did not change over recent decades, we can safely assume a fixed L and therefore that the relationship between estimated Δ18OLW and gs follows the scenario represented by the light blue line (i.e. a negative relationship), in line with our interpretation. Studies integrating tree ring δ18O data into land surface models (Keel et al., 2016; Barichivich et al., 2021) or using it as a proxy in paleoclimatological estimates in tropical regions (Evans, 2007), generally assume a fixed L-value; however, this did not prevent the interannual variability of tree ring δ18O chronologies from being reproduced. Using a fixed L-value, as applied in early demonstrations of the Péclet effect (see e.g. Barbour et al., 2004), will only affect the mean of the absolute modeled Δ18OLW values, but not the relationship between observed and measured Δ18OLW or Δ18OLW and evaporation rate, as shown in figs 3 and 4 in Barbour et al. (2004). Criticism 3: Although RH has an important effect on transpiration and, hence, on plant δ18O, we observed no systematic trends in humidity over most of our sites and interpreted this as evidence that the temporal trends in δ18O observed at some sites were not caused by trends in RH. Lin et al. (2022) challenged this, as well as our interpretation that the lack of a trend in Δ18OLW at other sites reflected the lack of a trend in gs. The authors reasoned that the potential for a high degree of interannual variability in RH and the absence of δ18O data for atmospheric water vapour increased the possibility that trends in Δ18OLW might have gone undetected. Although our study would indeed have benefited from direct measurements of water vapour δ18O, those data (as already discussed for δ18OP) have hardly been measured over the long-term. At high RH (which could be the case for the northeastern US sites in Guerrieri et al., 2019), back-diffusion of water vapour inside leaves can further complicate the interpretation of the seasonal dynamics of Δ18OLW (Lehmann et al., 2018), which was not the goal of Guerrieri et al. (2019). Nevertheless, a recent analysis provided strong evidence that RH has a much greater effect than water vapour δ18O on Δ18OLW (Cernusak et al., 2022). As such, Lin et al. (2022)’s criticisms are speculative and do not offer counter-evidence that would cause us to alter our interpretation. Based on their criticisms, Lin et al. (2022) concluded that our interpretations of δ18O measurements and their implications for trends in gs cannot be accepted with certainty. We fully agree but assert that our interpretation is reasonable and consistent with the evidence we presented in Guerrieri et al. (2019) and here. Our goal was to apply a novel approach (integrating tree ring isotopes, ecosystem fluxes and modelling) to probe the mechanisms of rising forest WUE and to offer the most plausible interpretation of the available evidence. We are grateful to Lin et al. (2022) for highlighting areas of uncertainty that require future attention and would welcome additional evidence that supports or counters our hypotheses. To enhance the physiological interpretation of tree-ring δ18O, future studies should include in-situ observations of source water δ18O (i.e. precipitation and water vapour, which could easily be achieved using existing monitoring networks world-wide, e.g. Fluxnet, NEON, ICP Forests), and a complete characterization of the δ18OP pathway from soil through tree xylem (Barbeta et al., 2022) and from water vapour to leaf water (bidirectional diffusion – Lehmann et al., 2019; Kagawa, 2022) to tree rings under field conditions (both at intra- and inter-annual resolution). Recent progress in deriving precipitation isotope time series using machine learning or isotope-enabled general circulation models can help to reduce uncertainties related to source water δ18O input (Nelson et al., 2021). The interpretation of tree ring δ18O, however, should go beyond the instantaneous or daily scale assessment of Δ18OLW, and be integrated with whole tree transpiration data (e.g. through sapflow measurements, now available at the global scale thanks to Poyatos et al. 2020) and plant hydraulic traits underpinning water relations (e.g. Flo et al., 2021). We thank the anonymous reviewers for the positive and constructive comments on the earlier version of the manuscript. Open Access Funding provided by Universita degli Studi di Bologna within the CRUI-CARE Agreement. RG drafted the manuscript with input from SB and SVO. All authors revised and contributed to improving the final version of the manuscript.
更多查看译文
关键词
stomatal conductance,tree‐ring
AI 理解论文
溯源树
样例
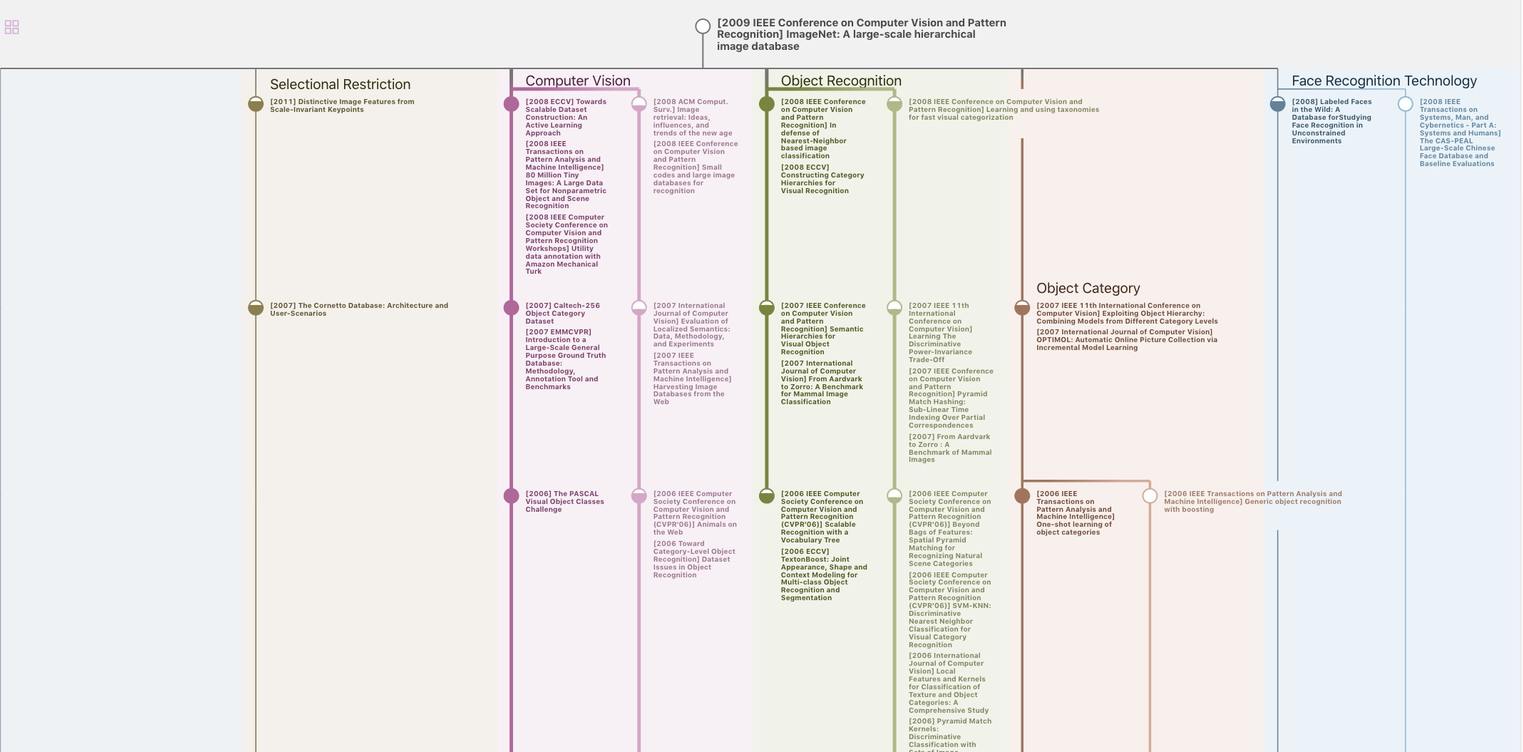
生成溯源树,研究论文发展脉络
Chat Paper
正在生成论文摘要